Javascript is currently disabled in your browser. Several features of this site will not function whilst javascript is disabled.
open access to scientific and medical research N-(9-Fluorenylmethoxycarbonyl)-D-phenylalanine
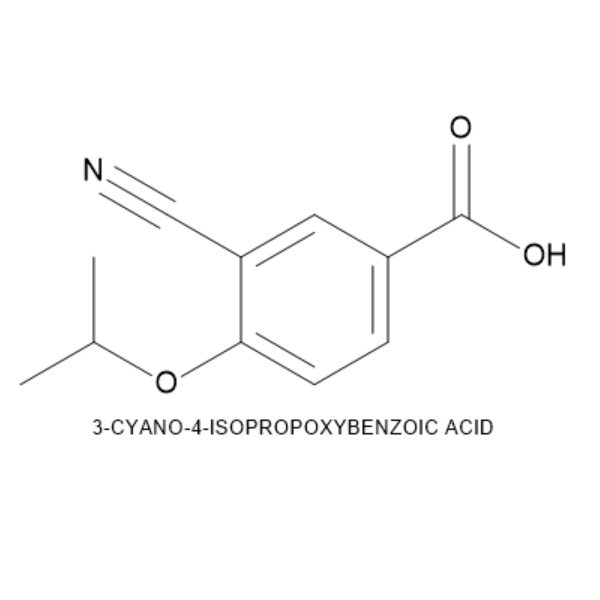
Open access peer-reviewed scientific and medical journals.
Dove Medical Press is a member of the OAI.
Bulk reprints for the pharmaceutical industry.
We offer real benefits to our authors, including fast-track processing of papers.
Register your specific details and specific drugs of interest and we will match the information you provide to articles from our extensive database and email PDF copies to you promptly.
Back to Journals » International Journal of Nanomedicine » Volume 19
Authors Peng C , Xu Y, Wu J, Wu D, Zhou L, Xia X
Published 4 January 2024 Volume 2024:19 Pages 109—135
DOI https://doi.org/10.2147/IJN.S441135
Review by Single anonymous peer review
Editor who approved publication: Professor Jie Huang
Cheng Peng, Yilin Xu, Jing Wu, Donghai Wu, Lili Zhou, Xinhua Xia School of Pharmacy, Hunan University of Chinese Medicine, Changsha, People’s Republic of China Correspondence: Xinhua Xia; Lili Zhou, Email [email protected]; [email protected] Abstract: The tumor microenvironment (TME) plays an important role in various stages of tumor generation, metastasis, and evasion of immune monitoring and treatment. TME targeted therapy is based on TME components, related pathways or active molecules as therapeutic targets. Therefore, TME targeted therapy based on environmental differences between TME and normal cells has been widely studied. Biomimetic nanocarriers with low clearance, low immunogenicity, and high targeting have enormous potential in tumor treatment. This review introduces the composition and characteristics of TME, including cancer‑associated fibroblasts (CAFs), extracellular matrix (ECM), tumor blood vessels, non-tumor cells, and the latest research progress of biomimetic nanoparticles (NPs) based on TME. It also discusses the opportunities and challenges of clinical transformation of biomimetic nanoparticles. Keywords: nanoparticles, biomimetic delivery system, tumor therapy, cell membrane-coating
TME refers to tumor cells and their microenvironment and is mainly comprised of tumor cells and their surrounding immune and inflammatory cells, tumor-associated fibroblasts and nearby interstitial tissues, tumor blood vessels, and various cytokines and chemokines, as shown in Figure 1. Figure 1 (A) shows composition of the TME: TME is a complex system, which is divided into cellular and non-cellular components. Cellular components include ECs, CAFs, TAMs, DCs, T cells, Regulatory T cells, B-cells, NK cells, MDSCs etc. Non cellular components include ECM and extracellular cytokines etc. (B) shows the composition of nano carriers modified by cell membrane. At present, cell membranes are mainly studied, including Erythrocyte, Tumor cell, Macrophage, NK cell, Neutrophils etc.
Figure 1 (A) shows composition of the TME: TME is a complex system, which is divided into cellular and non-cellular components. Cellular components include ECs, CAFs, TAMs, DCs, T cells, Regulatory T cells, B-cells, NK cells, MDSCs etc. Non cellular components include ECM and extracellular cytokines etc. (B) shows the composition of nano carriers modified by cell membrane. At present, cell membranes are mainly studied, including Erythrocyte, Tumor cell, Macrophage, NK cell, Neutrophils etc.
The biggest characteristic of TME is immunosuppression, and the ultimate goal of immunotherapy is to restore anti-tumor activity and weaken the immunosuppressive effect of tumor cells. Only by being familiar with the differences in the living environment between TME and normal cells can we use this as a starting point for safe and efficient treatment of tumors. However, the difficulty in treatment lies in the fact that, firstly, TME undergoes significant dynamic changes as the tumor develops. Secondly, there are normally expressed cells in TME, but due to its immunosuppressive properties, it is difficult to accurately target the tumor site and avoid potential adverse reactions caused by the impact on normal healthy cells in other tissues. Nanoparticles can deliver targeted anti-tumor drugs to anti-tumor sites through EPR effects and targeted molecules. In addition, nanoparticles can effectively improve the solubility and bioavailability of drugs, and reduce their toxic side effects. However, nanoparticles can be engulfed and cleared by the mononuclear phagocytic system as foreign substances, resulting in reduced efficacy. Biomimetic nanoparticles, on the other hand, successfully dress up as “in vivo components” by modifying cell membranes, exosomes, or other protein molecules to avoid clearance by the mononuclear macrophage system. This article first introduces the composition, characteristics, and targeted treatment methods of TME. Then, it introduces how biomimetic nanoparticles regulate the TME and their advantages in tumor treatment. Finally, it states the problems and challenges in clinical translation of nanodelivery systems.
As the microenvironment that tumors rely on for survival, TME includes CAFs, ECM, tumor vasculature, immune cells, etc. Unlike the environment in which normal cells survive, TME exhibits some unique characteristics to meet the growth and development of tumor cells, which can serve as research targets for tumor therapy, as shown in Figure 2 and Table 1. Table 1 TME-Related Durg or NPs Against Cancer Figure 2 Mechanism of therapy for cancer. The composition of TME is complex, with tumor promoting and tumor inhibiting components. Reversing the tumor immunosuppressive microenvironment requires enhancing anti-tumor cell activity (such as enhancing CTLs, DCs, NK cells, T cells, converting M2-TAMs to M1-TAMs) and weakening the influence of immunosuppressive components (such as weakening Tregs and MDSCs activity).
Table 1 TME-Related Durg or NPs Against Cancer
Figure 2 Mechanism of therapy for cancer. The composition of TME is complex, with tumor promoting and tumor inhibiting components. Reversing the tumor immunosuppressive microenvironment requires enhancing anti-tumor cell activity (such as enhancing CTLs, DCs, NK cells, T cells, converting M2-TAMs to M1-TAMs) and weakening the influence of immunosuppressive components (such as weakening Tregs and MDSCs activity).
CAFs are the most essential stromal cells in TME, accounting for 50% of the total tumor cells.23 The CAFs are differentiated from normal fibroblasts recruited and activated by secreted growth factors such as transforming growth factor β (TGF-β) and platelet-derived growth factor (PDGF) by tumor cells.24 CAFs significantly change morphology and functional protein expression compared to normal fibroblasts in the resting state.23 Morphologically, CAFs are spindle-shaped and relatively large, with various contractile and tension filaments in the cytoplasm and an abundant rough endoplasmic reticulum. Regarding functional protein expression, α-smooth muscle actin (α-SMA) and vimentin are highly expressed on the surface of CAFs.25 In recent years, many research reports have clarified the relationship between CAFs and tumors, providing a theoretical basis for using CAFs as new targets for tumor therapy (Table 1).
Compared to tumor cells, the CAFs are genetically stable, less likely to develop drug resistance and have a lower risk of drug resistance and tumor recurrence. Secondly, disrupting the crosstalk between CAF and cancer cells can alter the microenvironment that promotes tumors and improve the efficacy of combined therapy.26 Therefore, combinational therapy targeting tumor cells and CAFs is envisaged as a novel strategy for improving treatment efficacy and overcoming resistance.27,28 Many anticancer drugs targeting CAFs have been in the pre-clinical research or clinical trials phase.29 These drugs mainly act by directly damaging the CAFs by targeting their specific surface molecules or inhibiting the secretion of oncogenic factors and the signaling pathways involved by CAFs, thereby inhibiting the proliferation, invasion, metastasis and drug resistance of cancer cells.28 However, due to different sources and phenotypes, CAFs play different roles in different tumors and stages of the same tumor. Therefore, the function of CAFs in different types and stages of cancer needs further exploration.30
The ECM is a macromolecular substance synthesized and secreted by cells and distributed on the cell surface or between cells, acting as a vital tissue barrier to prevent tumor cell metastasis.31,32 It is a highly specific 3D polymer network, providing physical support for cells and regulating cell functions.33 Like most ECMs, the core components of tumor ECM comprise collagen, elastin, fibronectin, laminin, proteoglycans, and glycoproteins.34 Structural proteins, such as collagen, elastin, and proteoglycans, serve the basic skeleton, forming the ECM fibrous reticular complex on the cell surface and directly or indirectly connecting to the cell surface receptors through fibronectin or laminin and other linker molecules.35 These components together maintain the stability of ECM. Disruption of ECM balance by overexpression or abnormal behavior of one or more components may translate into changes in tumor invasiveness. Collagen type I, fibronectin, and other ECM proteins are up-regulated in breast cancer. Different pathological types of breast cancer have their own characteristic ECM protein expression profile, which is related to the prognosis of breast cancer, suggesting that the changes in ECM expression profile play a decisive role in determining the fate of tumor cells and tumor development.36 The dynamic changes in ECM in tumors are not only reflected by changes in the expression level and relative composition of ECM proteins but also in the physical properties such as the spatial topology and ECM rigidity.37
Tumor ECM can be targeted in several ways, including cellular activity in the ECM, ECM remodeling enzymes, and structural or physical properties of the matrix (Table 1). ECM exerts a “soil” effect in the development of tumors, where the ECM is complex and changeable; it can influence angiogenesis, remodeling, immune regulation mechanism, etc. affecting the tumor development. Thus, ECM in tumors provides a new direction for anti-tumor treatment. Matrix remodeling and degradation can promote tumor invasion to different degrees. How to find a balance point to inhibit tumor invasion is worth exploring. At the same time, using the characteristics of tumor ECM and its different from benign cells provide a new diagnostic idea for the clinical judgment of the nature of the lesion.
Angiogenesis refers to the generation of new blood vessels from existing ones, including the vascular membrane degradation, the activation, proliferation and migration of vascular endothelial cells (ECs), new angiogenesis and other complex processes.38 Like normal cells, tumor cells need blood vessels to transport oxygen and nutrients and excrete metabolic waste products. Under normal physiological conditions, in a mature organism, the vascular system lies in a long-term resting state and undergoes transient angiogenesis only in the event of an injury.39 During malignant tumor progression, angiogenesis remains in a normal state.39,40 When the tumor grows, due to higher oxygen and nutrient demand, continuous new angiogenesis progresses to fulfill the metabolic demand of tumorous cells, thus, making angiogenesis a process of pivotal importance for tumor growth and metastasis.41
Blood vessels in tumors are also structurally different from those in normal tissues.42 ECs are neatly arranged in normal tissues, where pericytes (PCs) are closely bonded to ECs.43,44
Vascular-targeted therapy strategies are mainly focused on inhibiting key signaling pathways that promote tumor angiogenesis, down-regulate the expression of vasoactive factors, inhibit tumor angiogenesis, cut off tumor feeding, and curb tumor growth, recurrence and metastasis45,46 (Table 1).
Based on the continuously revealed anti-angiogenesis mechanisms and related targets, researchers focused on ECs, PCs, basement membrane and other vascular components, and employing chemical modification, supramolecular self-assembly and other strategies to develop nanodelivery systems to improve delivery and anti-angiogenesis efficacy.47 In addition to long circulation and good permeability characteristics for improving payload delivery, it can integrate various functional components to actively target tumor blood vessels, improve hypoxia in TME, and regulate immunity48 (Table 1).
Immune cells are the main components of non-tumor cells in TME, including tumor-associated macrophages (TAM), dendritic cells (DCs), T-cells, B cells, natural killer cells (NK cells), neutrophils, and marrow-derived suppressor cells (MDSCs).49 Normal immune cells have a powerful anti-tumor effect and can induce innate and adaptive immune responses. However, due to the accumulation of immunosuppressive cells, cytokines or related components in the TME,50 immune cells become ineffective. Even the unique conditions of TME may transform some of these immune cells into tumor-promoting cells. Compared to directly targeting cancer cells, targeting TME has significant therapeutic advantages, as cancer cells are prone to developing drug resistance due to their genomic instability, while non-tumor cells in TME have more stable genetic properties.51
TAM is one of the crucial members of the innate immune response in TME,14 with dual antitumor properties, M2-TAMs that promote tumor development and M1-TAMs that inhibit tumor progression. In addition, Macrophages act as tumor inhibitors in the early stages of tumor development. Still, following tumor formation, TAMs perform the function of immunosuppression, promoting angiogenesis (secreting vascular endothelial growth factor (VEGF)52 and interleukin (IL)-8)53 and assisting tumor metastasis.54,55 Such as: facilitating Treg development and express elevated levels of immune checkpoint ligands, such as programmed death-ligand 1 (PD-L1), programmed death-ligand 2 (PD-L2) and inhibiting Cytotoxic T lymphocytes (CTLs) and NK cells and significantly exacerbating the immunosuppression effect.56 With the deepening of research, it has been found that TAM mainly plays a role in promoting tumors and immunosuppression. Therefore, targeted treatment plans for TAM mainly include: inhibiting the recruitment of tumor-related macrophages, promoting their failure and promoting M2-TAMs transformation into M1-TAMs can enhance macrophage antitumor activity (Table 1).
Bone marrow-derived DCs are the most potent antigen-presenting cells (APCs),55 which have the identification ability of the intruded pathogens via highly expressed toll-like receptors (TLRs). Following pathogen capturing, the major histocompatibility complexes are combined with T-cell surface molecules to activate them and initiate cellular immunity. The DC1 is the mainstay of exhibiting cross-presenting antigen. Moreover, activated DCs secrete tumor necrosis factor (TNF)-α, IL-1B, IL-12, and other pro-inflammatory factors to help the T-cells.57 However, these roles are limited to mature DCs, while immature DCs would promote tumor development and growth. The shock protein, TNF, IL-1, IL-6, inflammasome, TLRs, reactive oxygen species (ROS)13 and other factors have been found to promote DCs maturation58 while inhibited by Tregs. The VEGF, IL-10, and TGF-β in TME would affect DCs function, ie, hinder DCs maturation, and ultimately inhibits T-cell function. Huang et al reported that WNT2 secreted by CAFs would inhibit DC-mediated antitumor response.59
DCs activity is limited by a large number of immunosuppressive factors in TME and may not function even in the presence of a large number of antigens. Thus, the targeted treatment methods for DCs mainly include increasing DCs activity and increasing DCs recruitment (Table 1). The advantage of NPs is that they can specifically deliver antigen and adjuvant modified NPs to DCs, promoting DCs maturation and CTLs activation through antigen presentation or adjuvant assistance.
The CTLs, also known as CD8+T cells, are the center of the immune response. They recognize cancer cells by MHC-I class-specific antigen peptides to produce cytosolic particles, which exert a cytotoxic effect on the cancerous cells. Cheung et al found that macrophage-derived progranulin (PGRN) could inhibit MHC-I expression of cancer cells.60,61 In addition, the Kallikrein-related peptidases (KLKs), INF-1 and heme oxygenase-1 (HO-1) can also regulate CTLs activity.62 The CTLs exert an anti-tumor effect by secreting IFN-γ, which can up-regulate the expression of PD-L1 or PD-L2 in multiple cells, which could inhibit the anti-tumor effect of CTLs.52
The Treg refers to T cells expressing FoxP3.63 Under normal circumstances, it helps the body to avoid the occurrence of autoimmune diseases, but excessive Tregs in the TME can inhibit the body’s normal anti-tumor response. The large amount of Tregs in tumors can affect the prognosis of various types of cancer patients.64 Related studies have shown that Tregs are affected by αvβ8 integrin, where in their absence, Tregs cannot exert their inhibitory effect on T-cells.65 And IL-2 is an important growth factor regulating Tregs activity, Tregs need to capture exogenous IL-2 to survive, but recombinant human interleukin-2 (RIL-2) can activate NK and specific T-cells, in addition to activating Treg. Therefore, low-dose IL-2 ultimately leads to immune suppression, which can be achieved by targeting depleted Treg cells, injecting a large amount of IL-2, or inhibiting Treg activity through mutations in the Foxp3 gene (Table 1). However, the results of clinical trials were disappointing since large doses of RIL-2 were associated with severe systemic adverse effects, and making nanoparticles for local direct injection administration can concentrate RIL-2 in tumors, spleen, and lymph nodes and prevented RIL-2 from dispersing through systemic circulation to the thymus gland (the main site of Treg development), avoid causing autoimmune diseases.22
The adaptive immune cells comprise T and B-cells. Though researchers have been mainly focusing on the anti-tumor effects of T-cells in recent years, B-cells have been found to affect tumors significantly. After being stimulated by tumor-associated antigens (TAAs), they produce antibodies and granzyme B63 or activate T-cells as APC to exert tumor inhibition effect. Compared with B cells in peripheral blood, there is a difference in the expression of surface markers of B cells in tumor TME, with co-stimulating proteins (such as CD86) upregulated and CD23 expression reduced.63 The most important thing is that different B cell phenotypes directly or through interactions with other immune cells cause immune stimulation or tumor promoting effects, so it is necessary to comprehensively understand the mechanism of action of B cells.66
NK cells as one of the critical members of the innate immune system, unlike T cells or B cells have an innate immune response.67 Without antigenic stimulation, antitumor effects are performed through IFN-γ,52 perforin, and granzyme B.68 However, tumor cells or tumor-related cells will produce interleukin-6 (IL-6), IL-10, and TGF- β, prostaglandin E2 (PGE2) and other cytokines directly or indirectly inhibit the activation of NK cells. The scavenging capacity of NK cells is influenced by the signal transduction of NK cell surface activating receptors (CD16, member of NK-2 group D (NKG2D)), and inhibitory receptors (CD94, NKG2A, and killer cell immunoglobulin-like receptors (KIRs)),57 which is utilized by tumor cells in blocking the anti-tumor activity of NK cells. Injecting cytokines that activate NK cells or blocking the inhibitory effect of tumor cells on NK cells is a potential tumor treatment method.69
The secretion of G-CSF and GM-CSF by tumor cells affects the differentiation process of granulocyte macrophage progenitor cells, ultimately leading to the accumulation of MDCS in TME.70 MDSC effectively promotes tumor growth and metastasis through various immunosuppressive mechanisms, including metabolite depletion, upregulation of ROS, and secretion of various cytokines, as well as various non-immunosuppressive mechanisms, such as epithelial mesenchymal transition (EMT), promotion of tumor cell dryness, and tumor angiogenesis.71 And research has found that MDSC prevents immune checkpoint inhibitors from entering or in small amounts into TME, interfering with the action of ICIs. Secondly, other cells in TME can affect the infiltration of MDSC in TME (Table 2). MDSC is influenced by multiple factors, and the most crucial one is the differences in morphology and phenotype between human and mouse MDSC. Currently, most research is based on mouse models, and further exploration is needed to determine whether treatment methods can be successfully applied in clinical practice. Table 2 Cross-Talk Between Other Cells and MDCS in TME
Table 2 Cross-Talk Between Other Cells and MDCS in TME
In the process of targeted TME treatment, TME is dynamically changing under the influence of multiple factors. Only targeting tumor cells or other cells in TME alone is not sufficient to reverse or reshape TME, and multi target synergistic effects are needed. For example, Hei designed a CD47 antibody, a PD-L1 antibody modified with ROS-responsive chemical bond liposomes (CAR@aCD47/APDT1-SSL) loaded with carvedilol (CAR). The CD47 antibody was first released in response to ROS to promote macrophage phagocytosis and trigger a series of quasi-immune responses. The PD-L1 facilitated blockage of CTLs inhibition by cancer cells and resulted in a strengthened immune response. In comparison, CAR was released to inhibit tumor angiogenesis. The results showed that CAR@aCD47/APDT1-SSL improved TME by promoting macrophage phagocytosis, blockage of the PD-1/PD-L1 signaling pathway, and increasing CTLs infiltration, reducing Treg and M2-TAMs infiltration.72
Compared with traditional dosage forms, nanomedicines are known for their versatility and precision in cancer treatment, and have been studied for many years. Nanoparticles passively target the tumor site due to the EPR effect, and the gap between endothelial cells in TME increases vascular permeability, making it easier for nanoparticles to penetrate the tumor site. In summary, NPs have inherent advantages of high drug loading, elastic physical and chemical properties, flexible modification and an organic combination of multi-modal therapies, which make them suitable for loading with anti-tumor drugs, improving drug solubility, stability, pharmacokinetics, etc. They are widely employed in the pharma-medical industry.
Although nanoparticles can improve the tumor immune suppression microenvironment, they are synthesized and can be used as foreign substances, which are taken up in large quantities by the mononuclear phagocytic system (MPS) and quickly cleared, resulting in a decrease in drug delivery efficiency. Most immune cells are members of the mononuclear phagocytes system, including macrophages, monocytes, and conventional dendritic cells (cDCs). Therefore, controlling the clearance of nanoparticles by the MPS can significantly increase the anti-tumor activity of nanoparticles. At present, there are some strategies, such as particle size being an important factor affecting clearance pathways, and nanoparticles with small particle sizes (<10nm) can be excreted from the urinary tract through renal glomerular filtration. Large particle size nanoparticles are quickly cleared from the blood to the spleen and liver through the monocyte system. In contrast, larger NPs have a higher accumulation rate due to their longer intermediate cycle time, making them the best delivery carriers. In addition, surface modification of nanoparticles is a commonly used strategy for achieving immune escape, prolonging blood circulation, and enhancing tumor targeting. Surface modification with polyethylene glycol (PEG) is the most common strategy. Zhai et al prepared polymer micelles loaded with paclitaxel, consisting of PEG, 9-fluorenylmethoxycarbonyl (Fmoc), and IDO-1 selective inhibitor NLG919 (Dox/PEG Fmoc NLG). The pharmacokinetic parameters showed that compared with free DOX, the half-life (T1/2=14.4 hours) of Dox/PEG Fmoc NLG was significantly longer than that of the free Dox group (T1/2=0.85 hours), and the clearance rate (1.74 mL/h) was significantly lower than that of free Dox (9.83 mL/h), indicating that Dox/PEG Fmoc NLG has a longer blood circulation time. Flow cytometry results showed that compared with the Dox group, the infiltration of CD4+T cells, CD8+T cells, and M1-TAMs in tumors treated with Dox/PEG Fmoc NLG was significantly increased (p<0.05), while the infiltration of G-MDCS, M-MMDCS, M2-TAMs, and Treg was significantly reduced (p<0.05), which significantly inhibited tumor growth (p<0.05).73 However, research has shown that after the first injection of PEG modified nanoparticles, anti-PEG immunoglobulin M antibodies are induced, leading to rapid clearance of the nanoparticles from the bloodstream after the second injection of PEG modified nanoparticles. Secondly, modifying PEG chains increase steric hindrance, preventing the targeting molecules from binding to cell surface receptors, thereby reducing the uptake of NP by target cells. Rattan et al compared the clearance rate and cell-specific targeting of acetylated and polyethylene glycol modified dendritic polymers and found that the ability of acetylated modified dendritic polymers to reduce clearance rate was equivalent to that of polyethylene glycol, and polyethylene glycol reduced tumor specific targeting, while acetylation did not.74 Although different surface modifications of nanoparticles are beneficial for improving their biological activity, complex synthesis pathways can introduce risks. Better nanomedicine should not design nanoparticles from scratch but should obtain raw materials from biological systems. Many of the functions performed by cells are largely related to the biological macromolecules embedded in the cell membrane.75 Another strategy is to modify CD47 on nanoparticles and bind with signal regulatory proteins (SIRP) on phagocytes to inhibit the phagocytic ability of macrophages, ultimately avoiding the nanoparticles from being engulfed by macrophages.76,77 Biocoupling technology used to modify CD47 on nanoparticles may lead to protein denaturation.78 Therefore, CD47 simulated peptides can be used instead, but these peptides are different from SIRP α. The interaction between them is weak.76,79 Therefore, nanoparticles modified with biofilms or biomacromolecules can avoid clearance of the MPS. Therefore, nanoparticles modified with biofilms or biomacromolecules can avoid clearance of the MPS.
An ideal nanomaterial shall have the characteristics of prolonged systemic circulation and active targeting ability for improved cytotoxic drug efficacy in treating tumors. Since NPs are exogenous substances, they are easily recognizable by the body’s immune system, activating a cascade of downstream immune rejection, quickly cleared by the liver and kidney, which is the primary reason for poor drug delivery. In recent years, the biomimetic nanoparticulate drug delivery system has become a research hotspot that uses different biomimetic nanomaterials, including cell membranes, exosomes, lipoproteins, proteins and peptides, pathogens, etc. (Table 3). The NPs are either PLGA microspheres or magnetic nanocapsules, liposomes, silica, micelles, etc. Being biomimetic in nature, these nanomaterial delivery systems have the characteristics of good biocompatibility, targeting, and low immunogenicity and can protect drugs from degradation. Therefore, recently, various natural or artificial cell membrane NPs or nanovesicles have been developed and investigated for the treatment of cancer metastasis. Peptide-modified NPs actively targeting cancerous cells can improve drug delivery and increase safety. The subsequent sections focus on TME-related biomimetic NPs treating metastatic cancers. Their action for targeting and modulating the TME in local and distant metastatic regions was discussed. Table 3 Various Biomimetic NPs Against Cancer Metastasis
Table 3 Various Biomimetic NPs Against Cancer Metastasis
Membrane-coated NPs (M-NPs) comprise NPs coated with membrane components, including erythrocyte membrane, white blood cell membrane, tumor cell membrane and stem cell membrane, etc., showing actual cellular biological characteristics.
Erythrocytes are the most abundant cellular component of blood and remain with a life span of 120 days. Due to the absence of a nucleus and complex organelles in mature erythrocytes, cell membrane extraction and purification are convenient. Moreover, the erythrocyte membranes surface harbors multiple immunoregulatory proteins, such as CD47, C8-binding protein (C8bp), homologous restriction protein (HRP), CD59, etc., enabling them self-recognizable by the tissues and organs; hence, erythrocyte membrane can act as biomimetic, which can be used for coating of nanocarriers to prolong their circulation time and reduce rapid clearance. Rao et al used Fe3O4NPs as the core with nanocoating of erythrocyte membranes as a replacement for PEG. The results indicated significantly improved in vivo circulation time with reduced clearance of Fe3O4@RBC NPs by the RES, devoid of immune response induction both at MDSCs or the humoral level (immunoglobulin M and G (IgM and IgG)). RBCM@NPs is the first reported cell membrane biomimetic system and one of the most widely used natural carriers capable of resisting immune response and rapid clearance in biomedicine. As an in vivo drug delivery system, erythrocyte has excellent characteristics of high biocompatibility, long cycle half-life (~4 months), the membrane is flexible and stable, organelles lacking, enhanced carrying capacity and tumorigenicity (due to lack of nuclear DNA), and large surface area is conducive to surface modification.92
According to the characteristics of the disease and different drug delivery methods, drug platforms coated with erythrocyte membrane-coated NPs (RBCM-NPs) can be used in delivering chemical drugs, gene drugs, protein drugs, and other therapeutic agents to meet different needs. Studies have shown that RBCM-NPs can effectively deliver DOX to solid tumors and inhibit lymphoma growth, with significant in vivo safety and immune-compatibility. Since NPs’ enhanced permeability and retention are limited to angiogenesis at the tumor site, RBCM-NPs have relatively low tumor-targeting efficiency. Their specific applications can be further improved by modifying RBC-NPs with targeting ligands. In this study, Xie et al developed a novel invisible acoustic-sensitive NPs targeting complex coated with erythrocyte membrane (TXA+DOX/PFH/RBCM@cRGD), having a three-layer structure, where the inner layer comprised an aqueous phase with a high concentration of tranexamic acid (TXA) and sufficient DOX quantity to inhibit the fibrinolytic system and to eliminate tumor margin survival. The intermediate layer was composed of the perfluorinated hexane (PFH) phase, which can respond to low-intensity focused ultrasound (LIFU) radiation to produce acoustic phase transition and intense cavitation, resulting in a blasting effect, while the outer layer is comprised of erythrocyte membrane coating (RBCM) that help isolate the inner phase, prolong life cycle, and provide an assembly site for the targeted ligand (DSPE-PEG2000-cRGD). To verify its tumor-targeting activity and analyze its bioaccumulation characteristics, results were collected when the average tumor volume reached 500 mm3. For this purpose, equal molar amounts of CrGD-modified NPs (TXA+DOX/PFH/RBCM@cRGD) and unmodified NPs (TXA+DOX/PFH/RBCM) were respectively injected into the caudal vein of melanoma C57BL6/J mice model using B16F10 (ATCC® CCL-6475™). Following 6 hours of administration, the accumulation difference between TXA+DOX/PFH/RBCM-DiR@cRGD and CrGd-unmodified group was significant, with 20.55 folds higher average fluorescence intensity in tumor area compared to the CrGd-unmodified group, which indicated that compared to TXA+DOX/PFH/RBCM-DiR, the TXA+DOX/PFH/RBCM-DiR@cRGD had an efficient and long-lasting drug accumulation at the target site. The hematoxylin & eosin (H&E) and Masson trichrome staining showed that TXA was released after rupturing of TXA+DOX/PFH/RBCM@cRGD successfully inhibited the fibrinolytic system. The TXA preferentially binds to the k domain of plasminogen, completely blocking the binding of plasminogen or plasminogen heavy chain to fibrin, thereby inhibiting fibrin degradation, maintaining and stabilizing the skeletal structure of the fibrin reticulum. The TXA+DOX/PFH/RBCM@cRGD can effectively inhibit tumor intravascular fibrinolysis system, prolong thrombus state duration, and aggravate vascular tumor necrosis, destroying main tumor nutrient supply channels, eventually leading to rapid and extensive tumor necrosis and apoptosis.91 Liu et al designed an intelligent and effective nanosystem for synchronous radio and anti-angiogenesis therapy of cancer by combining ultrafine selenium NPs with bevacizumab (AvastinTM, Av). The nanosystem was coated with RBCM, which effectively extended the blood circulation time and reduced the elimination of the nanosystem by the autoimmune reaction.93
Recently, RBC-NPs have been widely used in phototherapy (PTT) and PDT. Liang et al coated black quantum dots (BPQDs) with RBCM to form a nano-vesicular (BPQD-RBCM-NV) bionic system. The BPQD-RBCM-NV mediated PTT combined with immune checkpoint blocking antibody (aPD-1) significantly increased the infiltration and activity of CD8+ T cells in tumors with extended circulation time, resulting in a significantly delayed growth of residual and metastatic tumors in vivo. The anoxic microenvironment within the tumor limits PDT therapy’s efficacy because it inherently requires oxygen conversion to ROS.94 To address it, Ren et al developed albumin NPs loaded with indocyanine green (ICG) and perfluorotributylamine, followed by their coating with RBCM to impart bionic properties. Due to the high oxygen capacity of PFCS, self-enriched NPs can enhance PDT by producing more singlet oxygen (1O2). After a successful NPs coating with RBCM, the immune clearance of macrophages (RAW264.7) can be effectively reduced, blood circulation time can be significantly prolonged, and high accumulation can be achieved in tumors.95
In addition to erythrocytes, tumor cells can be used as membrane sources for formulating biomimetic nano-drug delivery systems. These cells have infinite proliferation capacity and can be effectively cultured in vitro, and a large quantity of membranes can be isolated. Tumor cell membranes contain cell adhesion molecules on their surface, including cadherin, selectin, integrin, immunoglobulins superfamily (IG-SF), and lymphocyte homing receptors (eg, CD44). These receptors enable NPs coated with tumor cell membranes to escape immune clearance, exhibit homologous targeting behavior, and significantly improve their cancer-specific accumulation and retention abilities. These make them suitable for delivering tumor-targeted drugs and effective cancer treatment.
Chen et al studied the homologous targeting strategy of cancer cell membrane (CCM) NPs for targeted drug delivery by coating PLGA NPs with mouse breast cancer cell (4T1) membranes. The NPs loaded photothermal transduction agents (PTAs)-Prussian blue (PB), chemotherapeutic docetaxel (DTX) and immunoadjuvant imiquimod (R837). The western blotting showed that the homologous binding adhesion molecules (EpCAM and galectin-3) on the developed tumor cell membranes were further coated outside PLGA (M@P-PDR) nanospheres showed specific homologous adhesion to 4T1 cells through the homologous binding mechanism, with 61.67% cellular uptake, which was significantly higher than P-PDR nanospheres-treated group (12.54%). Under laser irradiation, combined with DTX, PTT induces in situ tumor clearance and releases TAAs, further enhancing the tumor cell’s immunogenicity. Similar to the in vitro results, the M@P-PDR showed a more vital ability to promote DC maturation with the aid of immune adjuvant R837, accompanied by increased secretion of cytokines (TNF-α, IL-6 and IL-12) in vivo. Furthermore, the release of DTX could also promote M2-TAMs into M1-TAMs conversion, which decreased from 68.57% to 32.80%, effectively alleviating the immunosuppressed TME, accompanied by a decrease in IL-10 level. Additionally, the homologous targeting of the nanospheres was improved through the coating of tumor cell membranes, and R837 and DTX were integrated into PTT to enhance immune response and alleviate immunosuppressive TME.96 Another study used poloxamer 407 (F127) coated with a human colorectal carcinoma cell line (HCT116) as a nanocarrier loaded with immune adjuvant R837. The developed F127-R837@M NPs were found to inhibit cancer cell growth by inhibiting angiogenesis.97
Cancer vaccine has been one of the research hotspots in recent years. Introducing a tumor vaccine to a patient can help overcome the immunosuppressive state caused by the tumor. Enhance immunogenicity activates the patient’s immune system, induces cellular and humoral immune responses, and controls or eliminates tumors. Gan et al developed CpG, a TLR9 receptor agonist-loaded aluminum phosphate NPs by reversed-phase microemulsion method, followed by their wrapping in B16F10 tumor cell membrane to obtain a cancer nano-vaccine. The developed nanocarrier had a size of 60 nm, which can be effectively drained into mouse lymph nodes by subcutaneous injection, significantly increasing the co-uptake of tumor antigen and CpG by lymph node resident APCs, promoting maturation of APCs, and enhancing lysosomal antigen escape. In cellular immunity, tumor cell membrane-coated aluminum phosphate NPs (APMC) were found to increase the level of CD8+ T and CD4+ T cells, especially CTLs, and induce the secretion of cytokines (IL-6, IFN-γ, and TNF-α). However, the anticancer immune response induced by APMC in mice was limited. Therefore, successful cancer immunotherapy must be combined with other sources to maintain the balance of the host immune system, such as PD-1 or PD-L1 checkpoint inhibitors.98
Li et al designed multifunctional biomimetic nanoplatforms (mEHGZ) in another study, combining starvation and immunotherapy. The platform was loaded with ICD inducer epirubicin (EPI), glucose oxidase (Gox), and hemin’s zeolitic imidazolate framework (ZIF-8). The NPs form the core, while 4T1 cell membranes comprise the shell. After ingesting mEHGZ NPs, Gox and hemin released by mEHGZ NPs can induce the Fenton reaction, promoting ROS generation and enhancing endoplasmic reticulum stress. Compared with EPI alone, the mEHGZ NPs enhanced the ICD effect. The antigens and danger-associated molecular patterns (DAMPs) released after ICDs can be used as nanovaccines to enhance tumor antigenicity and adjuvant, to promote DCs maturation and CTLs invasion, and activate tumor immune microenvironment. The tumor cells became sensitive to anti-PD-L1 antibody treatment in TME, confirmed in the 4T1 tumor-bearing mouse model, showing 82.02% of tumor growth inhibition rate after combined application of anti-PD-L1 antibody and mEHGZ, inhibiting lung tumor metastasis. Therefore, the nanosystem can be used as a nanovaccine to enhance the antigenicity and adjuvant properties of anti-PD-L1 antibodies and improve their therapeutic efficacy in cancers, including Triple-Negative Breast Cancer (TNBC).99
Stromal cells such as CAFs mediate many aggressive features of cancer and play a key role in cancer proliferation, invasion, metastasis, and angiogenesis. Two tumor cell membrane components (CCMFS) of U87 glioma cells and human breast cancer were selected to coat PLGA NPs forming CCMF-PLGA NPs. The results demonstrated that CCMF-PLGA NPs could actively reduce the ability of fibroblasts to attract cancer cells and reduce the ability of fibroblast-mediated tumor invasion and metastasis. At the same time, CCMF-PLGA NPs induced cancer-specific immune responses by observing the proportion of CD8+ and CD4+ T cells in the spleen and lymph nodes of immunized mice and the number of spleen cells producing INF-γ.100
Immune cells, also known as white blood cells, are colorless, nucleated blood cells, divided into granulocytes, monocytes and lymphocytes. They specifically include macrophages, neutrophils, CTLs, NKs, etc. The Immune cells can migrate freely in and out of the blood vessel, such as to the inflamed outer part of the blood vessel, to eradicate pathogens. They are widely distributed in the blood, lymph, and various tissues. They can recognize inflammation and accumulate in diseased areas, thereby affecting the progression of various diseases. Chronic inflammation is regarded as one of the main clinicopathologic features of tumors. Inflammation, which happens during tumor progression, allows immune cells to migrate to the tumor site. Studies have shown that macrophages or fibroblasts recruited by tumors may contribute to tumor growth and promote the development of metastasis and angiogenesis. Thus, selective blocking of the lymphocyte function-related antigen-1 or chemokine receptors (CXCR1 and CXCR2) on the white blood cell membrane significantly inhibited recruitment of leukocytes. A large number of chemokines are overexpressed in tumor tissues, and there are a large number of ligand–receptor interactions on the leukocyte membrane. Such as lymphocyte function-associated antigen 1 (LFA-1), macrophage-1 antigen (Mac-1), and P-selectin glycoprotein ligand-1 (PSGL-1).101 The NPs camouflaged with leukocyte membrane help NPs’ immune escape and active targeting ability and are widely used as drug delivery carriers.
Macrophages are derived from monocytes and are the most plastic and versatile innate immune cells in the hematopoietic system. They render strong plasticity and heterogeneity and can be polarized into classically activated M1 and alternately activated M2 to maintain cell homeostasis after corresponding stimulation. The M1 macrophages participate in pro-inflammatory responses and release various pro-inflammatory cytokines such as INF-γ, IL-1β, and TNF-α to help the host defense against pathogens, which often occurs in the early stages of inflammation and tumor. In contrast, the M2 macrophages participate in anti-inflammatory response and secrete IL-4, IL-10, IL-13, and other anti-inflammatory cytokines to promote inflammation regression, tissue repair, and wound healing, and often appear in the early stage of wound healing and the advanced stage of the tumor. M1-type macrophages are mainly used as drug carriers to enhance inflammatory response. Chen et al developed NPs coated with tumor-related macrophage cell membranes (NPR@TAMs), which can bind to CSF1, an immunomodulator targeting endogenous TAMs, and selectively accumulate in TME, which can eliminate the growth of primary tumors and inhibit effects in distant tumor growth. This TAMs membrane-based PDT− immunotherapy approach offers a novel strategy for personalized tumor therapy.102
Macrophages also contain a powerful lysosomal system, which can deal with foreign bodies in a fixed or free state, eg, phagocytosis and digestion of cell debris, pathogens, and cancer cells. When conventional NPs enter the body, they are ingested as foreign substances by the RES or MPS, being part of the immune system and consisting of monocytes, macrophages in the lymph nodes and spleen, and phagocytic cells such as Kupffer cells in the liver, resulting in their destruction or clearing from the systemic circulation. Currently, the most common strategy for reducing RES absorption is to shield NPs with PEG or other polymers, which is effective but only partially avoids ingestion. However, when NPs are coated with immune cell membranes, especially macrophage cell membranes, the RES system can be bypassed entirely since the immune system will recognize NPs camouflaging by macrophage cell membranes as “own” rather than “foreign”. Cao et al developed paclitaxel albumin NPs wrapped in macrophage cell membranes and found that macrophage cell membranes could significantly enhance the uptake of NPs by melanoma cells (B16F10) while reducing the uptake of biomimetic nanopreparations by macrophages, indicating that the bionic nanopreparations had good targeting and immune escape ability in melanoma cells.103 Similarly, Huang et al developed CrGd-modified macrophage membrane-coated nanovesicles to co-deliver METTL14 and TLR4 exciters to achieve the dual purposes of tumor inhibition and TME remodeling.104
A large number of macrophages exist around glioma, and PD-L1 lymphocytes, which are overexpressed on the cell membrane of TAMs, are combined with PD-1 lymphocytes expressed by tumor-infiltrating T lymphocytes (TILs) to induce T cells apoptosis and depletion further, inhibiting the activation of CTLs and trigger the immune escape of tumor cells. Yin et al reported macrophage membrane-coated nanoplatforms with enhanced PD-1 expression (PD-1-MM@PLGA/RAPA). The in vitro and in vivo GBM models demonstrated that PD-1-MM@PLGA/RAPA effectively crosses the blood–brain barrier in response to TME recruitment, while NPs accumulated at the tumor sites.105
NK cells are CD56+ lymphocyte subsets different from T and B lymphocytes, belonging to non-specific immune cells, which can recognize and kill tumor cells without a complicated immune response. In antitumor immunotherapy, NK cells can induce the polarization of proinflammatory M1-macrophages and target tumor cells through activated receptors (such as NKp30, NKp44, NKp46, DNAM-1 (CD226), NKG2D, RANKL, etc.) present in NK cell membranes.
Pitchaimani et al isolated an activated NK cell membrane with receptor protein (CD56) from NK-92 cells and fused it with cationic liposome to form NKsome. The engineered NKsome successfully retained the targeting proteins associated with the NK cell membrane on its surface, showing good biocompatibility, higher affinity to tumors than normal cells, improved in vivo tumor homing efficiency, and was able to target tumor cells more effectively under in vitro and in vivo conditions. The DOX-loaded NKsome also showed good antitumor activity against human breast cancer cells MCF-7 in vitro and in vivo.106
NK cells can target tumor cells and regulate immune response through receptors present on NK cell membranes. Based on this, Deng et al used NK cell membrane-coated mPEG-PLGA NPs and photosensitizer (TCPP) to achieve combined immune and photodynamic therapy. The NK-NPs can induce pro-inflammatory M1-macrophage polarization through interacting NK cell membrane proteins such as RANKL or CB1 with macrophage surface receptors such as TNF receptors or TLRs-4, resulting in cell membrane immunotherapy. Meanwhile, NK-NPs loaded with TCPP can directly eradicate primary tumor cells through PDT and activate APCs through PDT-induced ICD. These dying cancer cells secrete damage-related molecular patterns (DAMPs), promoting DCs activation and initiating an adaptive immune response, thereby improving the anti-tumor immune efficiency of the NK cell membrane. Therefore, this approach offers a promising strategy for tumor immunotherapy.
Neutrophils are the most abundant (accounting for 50%~70% of all white blood cells) and the most important immune white blood cells in the human body, housing a large number of bactericidal molecules, proteases, proteins, with the role of clearing pathogens and resisting exogenous infections. Tumor-associated neutrophils (TANs) are neutrophils recruited into tumor tissues by chemokines in the TME. They play a role in various ways in tumor occurrence, development, and metastasis. Neutrophils are different from other white blood cells in that they are not restricted to specific circulation areas and are free to travel through blood vessel walls and body tissues to attack invaded antigens.
The adhesion molecules on the surface of inflammatory neutrophils have been shown to target circulating tumor cells (CTCs). Thus, NPs camouflaged by neutrophil-cell membranes can retain their biological binding properties. Targeted CTCs, where NPs were coated with neutrophil membranes, can effectively treat cancer metastasis. When loaded with second-generation proteasome inhibitors (carfilzomib), the biomimetic NPs selectively consume circulating CTCs, preventing early metastasis and inhibiting already occurred metastasis.
In another study, Cao et al was able to overcome the blood-pancreatic barrier and achieve pancreatic-specific drug delivery in vivo by coating celastrol-loaded PEG-PLGA NPs with neutrophilic cell membranes (NNPs), which demonstrated significant tumor inhibition in both in situ and ectopic tumor models, which significantly extended survival and minimized liver metastasis in tumor-bearing mice.107
Zhao et al developed gemcitabine-loaded neutrophil cell-wrapped liposome NPs (NE/Lip-GEM) and studied their use as complementary drugs for nanosecond pulsed electric field (nsPEF) therapy. The results showed that NE/Lip-GEM accumulated in ablated tumors and significantly improved the efficacy of nsPEF therapy due to nsPEF ablation activating the release of inflammatory cytokines at the tumor site, promoting the migration of neutrophils to the ablation site.108 In addition, Zhang et al developed PLGA NPs coated with neutrophil cell membranes (NM-HB NPs), which were found to promote ROS production and mitochondrial dysfunction by inhibiting the expression of the pro-apoptotic protein JUNB. They elaborated on the binding mechanism of NM-HB NPs and PDT. These results suggested that NM-HB NPs can be used not only as a new therapeutic agent for HCC but also for efficient near-infrared imaging.
Platelets have no nuclear structure, and their cytoplasm contains a variety of organelles and platelet particles, including mitochondria, lysosomes, endoplasmic reticulum, α-particles and dense particles. They are important in vivo circulating cells and have been widely used in developing bionic drug delivery systems. The abundant membrane proteins distributed on the surface of platelets can play specific physiological functions under the mediation of related proteins, eg, integrin protein αIIb is expressed on the membrane surface of platelets and tumor cells, mediating their mutual adhesion with plasma fibrinogen and hemophilia factor. The P⁃ selectors on the platelet surface can be mutually recognized with CD44 receptors on the tumor cell surface, thus enabling platelets to target multiple tumor cell lines, promote intravascular migration and adhesion of tumor cells, colonizing and growing at secondary sites, thus affecting the occurrence and development of tumors. Using this property, Wang et al coated bufalin-coated chitosan-polylactic acid-glycolic acid NPs with platelet membrane, indicating that more PLTM-CS-pPLGA/Bu NPs were significantly bound to H22 liver cancer cells compared to uncoated CS-pPLGA/Bu NPs, due to the P-selectin targeting on the surface of platelets binding to the CD44 receptor of H22 liver cancer cells. It also inhibited tumor growth more effectively than other bufalin preparations.109
Platelets target tumor cells and modulate immune responses through the self-recognition properties of the platelet membrane. Jiang et al developed biomimetic magnetic nanoplatforms coated with platelet membranes (Fe3O4-SAS@PLT) and indicated that Fe3O4-SAS@PLT not only effectively initiates iron-induced death of tumor cells but also can facilitate the conversion of immunosuppressive type M2 to anti-tumor type M1 macrophages, inducing an effective immune response, and enhancing the therapeutic effect of PD-1 blockers in vivo.110 By binding anti-PDL1 antibodies (anti-PDL1) to the platelet membrane surface, Wang et al reported that it could promote the delivery of anti-PDL1 antibodies to tumor sites and targeted CTCs, thus inhibiting tumor recurrence and metastasis after surgery.111
In addition, platelet-coated NPs can also target vascular injury sites through receptor–ligand interactions between platelet membranes and tumor vascular endothelial cells, while tumorous vessels provide nutrients and oxygen to tumor tissues and remove metabolic waste, which is vital to tumor proliferation and metastasis. Li et al coated platelet membranes on mesoporous silica (MSN) NPs loaded with vascular disruption agents and antiangiogenic drugs, which showed avoidance of early systemic clearance and target precisely to ruptured blood vessels, along with good antitumor effects in xenograft liver tumor mouse models.112 By taking advantage of the acidic microenvironment at the tumor site, some researchers designed acid-sensitive nanocarriers coated with platelet membranes to enhance the tumor-targeting ability, improve biocompatibility, and effectively consume lactic acid and relieve hypoxia, thus improving the therapeutic effect of the tumor under the combined action.113
In recent years, with the continuous development of membrane biomimetic technology, more and more research has focused on synthesizing hybrid membrane biomimetic NPs by fusing two different cell membranes from different sources to achieve the organic combination of different biofilm functions. The selection criteria of cell membranes mainly depend on the unique characteristics of different cells and the disease treatment needs. Zhang’s group reported NPs coated with an erythrocyte-platelet mixed membrane, having surface membrane protein markers from both types of cells; the resulting double-membrane coated NPs exhibited good long circulation and distribution in mouse models. Compared to single RBCM-coated NPs and platelet-coated NPs, the hybrid membrane-coated NPs showed the crossover characteristics of the two single-membrane-coated NPs. Therefore, hybrid membranes, including erythrocyte-tumor cell hybrid membranes coated, platelet-leukocyte hybrid membranes coated, and tumor stem cell-platelet hybrid membranes coated NPs, are advocated in targeted tumor therapy.114
Gong et al designed pH-sensitive PLGA NPs based on the fusion of macrophage (RAW264.7) and breast cancer cell (4T1) membranes. The NPs were developed from the immunometabolic regulator metformin (Met) and targeted fibrin protein-1 mRNA (siFGL1). Under transmission electron microscopy (TEM), the MC-PLGA@Met-CO2/siFGL1 NPs showed a core-shell bimolecular membrane structure with a particle size of about 142 nm. The in vitro cell experiment results showed that the uptake level of MC-PLGA@Met-CO2/siFGL1 NPs in 4T1 tumor cells was significantly higher than that in the uncoated NPs group and not captured by lysozyme after 4 hours of administration, showing immune camouflage and tumor targeting abilities. In addition, the in vivo results indicated that the Met-loaded hybrid bionic membrane-camouflage PLGA NPs could effectively alleviate tumor hypoxia and induce M1 type differentiation of TAMs, thereby improving tumor immunosuppressive microenvironment.115 In another study, Gong et al coated this hybrid membrane on DOX-loaded PLGA NPs (DPLGA@[RAW-4T1] NPs) for treating lung metastases from breast cancer. The surface of DPLGA@[RAW-4T1] NPs was modified with RAW264.7 and 4T1 cell membrane proteins. Due to the high expression of α4 and β1 integrin, the addition of macrophage cell membrane significantly improved the targeting ability of DPLGA@[RAW-4T1] NPs to metastatic tumors, while 4T1 membrane can target homologous cancer cells, actively reaching metastatic lung site of the breast cancer. The in vivo lung metastasis model experiments in mice showed that DPLGA@[RAW-4T1] NPs tend to accumulate at the site of inflammation and are explicitly targeted at the breast cancer lung metastasis site. Meanwhile, DPLGA@[RAW-4T1] NPs showed excellent chemotherapy potential, with about 88.9% anti-metastasis efficacy. This study provided a promising approach for treating breast cancer metastases.116
Similarly, Wang et al designed pH-sensitive micelles based on the envelope of erythrocyte-tumor cell hybrid membranes (DH@ECm). In the acidic TME, micelles exhibited membrane escape effects to promote the recognition and interaction of TAMs. The in vivo testing showed that DH@ECm can reverse the tumor immune system and increase the number of CD8+T cells, with a 64.8% tumor suppression rate, immune camouflage and tumor targeting capabilities.117
The biggest advantage of nanoparticles coated with cell membranes is to reduce or avoid the clearance effect of RES. In addition, various types of cell membranes have their own advantages and disadvantages, such as poor targeting of red blood cell membranes to tumor cells, requiring additional modification of targeted molecules. Tumor cells, immune cells, and platelet membranes themselves have tumor targeting properties, so fusing different cell membranes can complement each other’s strengths, expand the targeting sites for tumor cells, and achieve multi-target and multi-directional collaborative treatment of tumors.
Proteins are one of the basic human body components and are multifunctional and biocompatible. Tumor cells have specific receptors on the surface. Natural protein-modified NPs can bind to them to achieve active tumor targeting and deliver drugs to tumors to exert anti-tumor activity.
Serum albumin is the most abundant protein in the blood. It has the advantages of a long half-life and preventing the degradation of NPs by cells, and it is widely employed as a nanocarrier for delivering drugs. However, the FDA-approved albumin-bound paclitaxel (Abraxane) has yet to achieve the desired effect in clinical treatment. Studies have shown that it was related to non-tumor cell components of TME.
Feng prepared palmitate-modified human serum albumin (THP) NPs loaded with pirarubicin (THP-PSA NPs), which targets the TAMs in TME to improve TME. The flow cytometry results showed that compared to the normal saline group, the proportion of TAMs in the TME was reduced by 60.9%, the number of MDSCs was reduced to 55.6%, and the expression levels of immunosuppressant cytokines (TGF-β1 and IL-10) were reduced by 77.6% and 66.5%, respectively. It has been demonstrated that NPs can effectively target and consume TAMs, reduce the recruitment of MDSCs, and inhibit the secretion of immunosuppressive cytokines.118
Ferritin (Fn) plays a vital role in iron storage and binds explicitly to transferrin receptor 1 during iron uptake. In normal cells, transferrin receptor-1 (TfR1) is expressed at a low level, but in tumor cells, the expression of transferrin receptor is significantly increased to meet the iron requirement of tumor cells.
Wang prepared Mn2+ and Lap mannose (Man) modified albumin/ferritin (Ft@Lap) cross-linked NPs, where ferritin can encapsulate Lap in the cavity through hydrophobic interaction and then bind to TfR1 to achieve targeted delivery of Lap. By binding to the TfR1 receptor, Ft@Lap was selectively endocytosed by tumor cells, inducing the immunogenic death of cancer cells and releasing a large number of DAMPs. In addition, the cGAS-STING pathway is important to initiate an antitumor innate immune response, and studies have shown that Mn2+ is a STING agonist. Therefore, BSA-Man@Mn2+ can be ingested by DCs through mannose, activate the cGAS-STING pathway, and jointly promote DC maturation, enhance T cell infiltration, and improve immune efficacy.119
Cancer cells in TME are different from normal cells in that they have unique metabolic patterns governed by enzymes. For example, MMPs can mediate proteolysis, continuously degrade ECM, promote epithelial–mesenchymal transition (EMT), and ultimately lead to tumor invasion and metastasis.120 The peptide sequences targeting MMPs can be modified onto NPs to improve the specific recognition of cancer cells, which is of great significance in improving the TME and efficiency of tumor treatment. Chen designed self-assembled NPs (TGMF) that can target and induce apoptosis of cancer cells. Selective cleavage of TGMF by a high concentration of MMP-2 in TME resulted in the release of GO-203, inducing death to cancer cells, which can block intracellular mucin 1 (MUC1) and disrupt the REDOX balance in cells. The results showed that TGMF could significantly reduce tumor volume and had a high survival rate (60-day survival rate, up to 40% (p<0.001)).121 In addition, TME-related enzymes and biomimetic peptides that mimic the effects of proteins or peptides in TME have also been developed to regulate TME. The PSAP is a proteolytic glycoprotein in late lysosomes, which can inhibit the expression and production of tumor protein thrombospondin-1 (TSP1), thereby inhibiting tumor metastasis.122 On this basis, Wang et al designed a cyclo-pentapeptide derivative-DWLPK (named PSAP peptide) for treating metastatic ovarian cancer. Results showed that PSAP peptide could recede metastatic tumors to the extent that the lesions were undetectable.123 Xiang et al designed a biomimetic peptide (SVS-1) to modify DOX-loaded N-(2-hydroxypropyl) methyl acrylamide (HPMA) polymer (SVS-1-P-DOX). Natural antimicrobial peptides (AMPs) can interact with cancer cell membranes and enter the cytoplasm but have a low affinity for normal cells, a potential carrier for targeting cancer cells. SVS-1 is a peptide mimicking AMPs, results showed that compared to free DOX, the blood circulation time and targeting ability of SVS-1-P-DOX were significantly improved, with a 78.7% tumor inhibition rate and with insignificant toxic and side effects.124
White blood cells can avoid the uptake of MPS and increase the circulating time in the body. On this basis, Roberto Molinaro et al prepared liposomes loaded with doxorubicin modified white blood cell membrane proteins, called leukocytes.101 The experimental results showed that at 24 hours, the accumulation of liposomes in the main MPS organs increased, with an increase of 1.6 times in the spleen and 4.4 times in the liver. Moreover, white blood cell bodies have good targeting ability for 4T1 breast tumors, and significant accumulation can be observed on the tumor-related blood vessel walls. When leukocytes act on B16 tumors, the accumulation of leukocytes in the tumor increases 9-fold compared to liposomes (p<0.0001). Secondly, this phenomenon has also been observed in breast tumors and melanoma tumors, indicating that leukocytes have targeting properties towards various solid tumors. Therefore, compared with free doxorubicin, delivering doxorubicin with leukocytes can significantly inhibit tumor growth.
Protein or peptide modified nanoparticles recognize and lock in tumor cells through a “ligand receptor” reaction, promoting the entry of nanoparticles into TME, improving the accuracy of nanoparticles delivery to tumor cells, enhancing tumor cell penetration, and reducing drug toxicity. In addition, peptides can serve as both targeted ligands and therapeutic bioactive agents.
Folate receptor (FR) is a glycoprotein on the cell surface that could combine with folic acid (FA) with high affinity. Folic acid receptor is highly expressed in breast, lung and kidney tumors and acts as an efficient tumor biomarker and is the most common target molecule with non immunogenicity, high stability, and good tissue penetration. Qing used FA-modified OMV@CaPs to treat refractory tumors, and results showed that the tumor enrichment rate of FA-modified NPs was increased by 1.6 folds, significantly increased levels of pro-immune cytokines (IFN-γ, IL-12 and TNF-α, P<0.01), reduced the tumor volume, with insignificant lung and bone metastasis. It shows that FA modification can increase the accumulation of NPs in tumor tissues and activate a more robust immune response.125 Nie et al prepared FA-modified DOTAP, MPEG-PCL-MPEG, and FA-PEG-PCL-PEG-FA self-assembled CKb11 plasmid-loaded nanodelivery system (F-PPPD). Experimental results showed that compared to non-targeted PPPD NPs, the F-PPPD significantly promoted CKb11 release (P<0.01), promoted M1 and inhibited M2 polarization, activated CD4+T and CD8+T (P<0.01), and reduced the MDSCs infiltration. More importantly, there was no significant difference in the expression of CKb11 in the heart, liver, lung and other organs after F-PPPD treatment, which avoided the adverse reactions caused by systemic administration of CKb11. In conclusion, after targeting tumor cells, F-PPPD promotes CKb11 secretion, activates T cells, inhibits M2-TAMs polarization, reduces immunosuppressive cell infiltration, improves tumor immunosuppressive microenvironment, and avoids excessive “immune enhancement” adverse reactions.126
Monoclonal antibodies (mAbs) are highly homogeneous antibodies produced by a single B-cell clone that target only a specific epitope. After the fusion of infinitely proliferating myeloma cells and B lymphocytes, a large number of monoclonal cell lines were obtained by screening and cloning. The highly homogeneous antibody against a specific antigen produced by the cell lines has the advantages of high sensitivity, high specificity and less cross-reaction. Lu et al prepared MnOx with multivalent Mn (Mn2+ and Mn3+) NPs that were modified with 3-aminopropyl-triethoxysilane (APTES) to bear amino groups for conjugation with T-cell antibodies (anti-CD3 and anti-CD28 mAbs, CD). The results showed that compared to unmodified mAbs, the CD-MnOx@CM NPs caused significantly higher pro-immune cytokines and increased CD8+T cell infiltration. The in vivo experiments showed that the number of metastatic nodules was significantly reduced (P<0.01) after the modified mAbs application, making tumor detection difficult. In addition, the MnOx@ CM NPs affected serum IFN-γ, but CD-MnOx@CMNPs exerted no effect on CD8+T cells in the spleen and cytokines in the serum, indicating that NPs increased the antitumor effect by activating local immunity.127
Tumor-penetrating peptides have both tumor-targeting and cell transmembrane functions. Sugahara found a cyclic peptide with CRGDKGPDC sequence (iRGD), which can actively target tumor cells with high expression of integrin αvβ3 receptor, and had a membrane penetrating effect. The mechanistic investigations revealed that the intact iRGD binds first to the surface of cells expressing integrin αvβ3 receptor and is proteolyzed to produce CRGDK fragment. CRGDK fragment had a low affinity for integrin αvβ3 receptor and a high affinity for neuropilin-1, which promoted the transfer of CRGDK from integrin αvβ3 receptor to neuropilin-1, resulting in the transmembrane activity. Song et al used modified staphylococcal enterotoxin C2 (ST-4) linked to iRGD cyclic peptide to improve the poor tumor targeting and penetration of ST-4. The experimental results showed that compared to the free ST-4 group, the inhibitory effect of ST-4-IRGD on B16F10 and 4T1MC was significantly improved (P<0.05), with a significantly increased (P<0.01) relative infiltration intensity of CD8+T cells and CD4+T cells. The in vivo imaging of the ST-4-iRGD group showed higher fluorescence signals with a wide fluorescence distribution range, which proved that ST4-iRGD had good in vivo targeting, penetration, and tumor tissue distribution ability.128
Aptamers are a class of single-stranded oligonucleotides with high specificity, strong affinity, and accurate target recognition, which are screened from random DNA or RNA libraries by ligand-enriched phylogenetic technology (SELEX). Aptamer has the advantages of simple synthesis, low molecular weight, high stability, low toxicity, controllable program, tumor penetration and high retention. Kim et al designed DNA aptamer-modified liposomes containing DOX and IDO1 siRNA (Aptm[DOX/IDO1]) in the same kit. The Aptm[DOX/IDO1] used aptamer-receptor binding to deliver drugs to cancer cells, released DOX, triggered ICD, induced aggregation of effector T cells in TME, inhibited IDO1 expression in combination with IDO1siRNA, inhibited Tregs activity, and ultimately reversed immunosuppressive TME, activated immune response, and killed the tumor cells. The results showed that compared with the liposome alone group, the number of effector T cells and mature DC cells in TME after Apt modification was significantly increased (P<0.01), with a significantly decreased (P<0.05) number of Tregs cells, and the expression of IDO1 was significantly decreased (P<0.01). Additionally, no metastatic tumors were observed in mice treated with Aptm[DOX/IDO1], which proved that Apt modification could achieve specific delivery and accumulation of drugs at tumor sites.129
Exosomes are bilayer liposomal vesicles secreted by living cells, which affect cell-to-cell communication, signal transduction and tumor metastasis, and can be secreted by mammalian and plant cells. Chen et al extracted exosome-like nanovesicles (TFEN) from edible tea flowers to treat breast cancer. The results showed that it could be absorbed by human breast cancer cells up to 94.4% and had good biocompatibility. Oxidative stress can induce mitochondrial damage and cell cycle arrest and then promote the death of tumor cells. The total concentration of ROS in MCF-7 and 4T1 cells treated with TFEN was 14.2 and 9.3 folds higher, while NO levels were 5.2 and 3.5 times higher compared to control cells, respectively. TFEN can activate oxidative stress in cancer cells but not normal cells, inhibiting tumor growth and metastasis in vivo. In conclusion, TFENs can activate oxidative stress in cancer cells, cause mitochondrial damage, and eventually cause the death of cancer cells. Compared with artificial NPs, natural exosome-like NPs have high biocompatibility, low cost, and are easy to mass production and clinical transformation.130
Tumor-derived nanovesicles (TNVs) have achieved good results as tumor vaccines in clinical trials, but their therapeutic efficacy is poor due to the immunosuppressive microenvironment. Hu designed DOX-loaded mixed liposome nanovesicles (DOX@LINV), which can promote APC maturation. The tumor-derived vesicles retain the ability to target cancer cell antigens or proteins, antigens in LINVs are recognized and phagocytosed by DCs, activating DCs and immune response. The uptake rate of mixed liposome nanovesicles by LLC cells and B16F10 cells were higher than DOX@LIP, which could induce a more substantial ICD effect. The in situ 4T1 tumor model showed a tumor inhibition rate of 80% for the DOX@LINV4T1 group, indicating a good tumor treatment effect.131
ECM is an important component of TME, providing transport routes and nutrients for tumor growth. The construction of artificial ECM has become one of the strategies for cancer treatment. Hu et al developed an NP that could transform laminin (LN) to mimic peptide-1 (BP-KLVFFK-GGDGR-YIGSR). After injection into the body, the NPs were targeted to the tumor by the EPR effect or RGD-YIGSR. After binding to the receptor on the surface of tumor cells, it was transformed into nanofibers and finally into aECM. They bind competitively to ECM, inhibiting tumor growth and metastasis.132 The changes in ECM structure can affect the metabolic response of tumors and interfere with tumor growth. Zheng et al used fibrinogen and prothrombin to accumulate in the blood vessels with minimally invasive oral tumors after other treatments, resulting in a coagulation reaction, blocking the nutrient source of aECM and tumor cell migration, and limiting tumor growth, with high effectiveness and safety in rodents.133 Theoretically, as long as the tumor blood vessel coagulation is triggered, the antitumor effect can be exerted, which provides a new way for targeted therapy, radiotherapy, chemotherapy, etc.
In the 19th century, Dr Willhem Busch discovered bacterial therapy, and since then, bacteria have attracted immense attention for their use in cancer treatment because of their autonomic drive, anaerobic, and ability to secrete cytokines to activate an anti-tumor immune response. Bacteria have natural advantages but also have the risk of suppressing the body’s immune response and damaging healthy human tissues. Based on this, researchers used gene modification and nano-drug delivery to increase bacteria potency and reduce toxicity. Combining bacteria and nanotechnology can preserve environmental sensitivity, tropism, motility, and low-oxygen growth conditions. It also realizes precise, controlled, and sustained drug delivery. The combination of bacteria and nanotechnology mainly includes bacterial cell-surface nanoengineering, bacterial intracellular nanoengineering, and NP-based bionic bacteria. Xing et al connected ICG to magnetospirillum magneticum (AMB-1) under an externally applied magnetic field that can be positioned and manipulated, providing a new way to target tumor therapy precisely. Vairavel et al reported that the intracellular synthesis of gold NPs utilizing Enterococcus could induce tumorous cell mitochondrial death, subsequently inducing the death of cancer cells. Bionic bacteria refers to extracting active components such as bacterial membranes and vesicles from natural bacteria as drug transport carriers.134 Selvanesan et al used Listeria to deliver antigens to tumor cells to activate memory T cells, and gemcitabine (GEM) was used to reduce the amount of MDSC and TAMs in the TME.135
Except for peptides or proteins as targeting molecules, monoclonal antibodies, aptamers, etc. can all be used as targeting molecules for tumor cells. Tumor derived exosomes have the natural advantage of targeting cancer cells and being able to fuse with tumor cell membranes. Bacteria have the characteristics of targeting tumor hypoxic sites, strong tumor permeability, and stimulating immune responses. Combining nanomaterials with bacteria can compensate for the shortcomings of low targeting efficiency and poor tumor penetration of nanomaterials. In summary, extracellular vesicles, bacterial therapy, and aECM can all serve as biomimetic strategies for targeting TME, in addition to nanoparticles modified with cell membranes or molecules.
Nanomedicine has potential advantages in overcoming biological barriers, effectively delivering hydrophobic drugs and biological products, and prioritizing targeting disease sites. However, due to many challenges and obstacles at different development stages, only a relatively small number of nanoparticles-based drugs have been approved for clinical use. FDA approved nanomedicines for cancer treatment include metal NPs (Aurimune), AuNPs, polymer drug conjugates (Eligard), SMANCS, (Lipid based Nanoformulations (Marqibo, Doxil), Recombinant virus (Gendicine), Drug Targeted Antibodies (Kadcyla) or herbal NPs (Nano formula curcumin), etc. obtain clinical trials of nanoparticle therapy for tumors in the past 10 years through https:///clinicaltrials.gov/ (Table 4). Transforming nanomedicine from a theoretical concept to clinical therapeutic value is challenging. The distribution and detection of nanomedicine in vivo are difficult to quantify. Although fluorescence or radioactive labeling methods can be used to obtain the distribution image of nanoparticles in vivo, labeled nanoparticles are prone to degradation, which may result in fluorescence or radioactive molecules not being able to label all nanoparticles, leading to incorrect results. Furthermore, it is unknown whether fluorescence or radioactive molecular labeling will alter the distribution of nanoparticles. The preparation method of nanoparticles is complex, and laboratory research has small-scale production with good reproducibility. However, after expanding production and manufacturing, the controllability of physical and chemical properties is crucial. The first nano drug approved by the US Food and Drug Administration, Doxil is an example that the production of this drug had to be suspended in November 2011 due to production and sterility issues. The problem was not resolved until 2014. The high cost of raw materials for preparing nanoparticles can affect the large-scale production of nanocarriers. In order to compensate for high costs, the clinical therapeutic effect of nanomedicines must be significantly superior to traditional anticancer drugs. The most important thing is to ensure the safety of nanomedicines. Assessing the in vivo and long-term toxicity of nanomaterials is difficult, and there are currently no evaluation standards. Nanomedical therapies can only be judged based on individual evaluations of benefits and risks, so the regulatory process is time-consuming. In the future, detailed characterization and quality control guidelines for nanomedicine products are needed. Table 4 Clinical Trials of Nanoparticles for Treatment of Tumors in the Past 10 Years
Table 4 Clinical Trials of Nanoparticles for Treatment of Tumors in the Past 10 Years
In this review, we summarized the differences between TME and normal cell microenvironment, as well as the therapeutic targets targeting TME. So far, CAFs, ECM, tumor blood vessels, immune cells, etc. are all effective targets for treating tumors. The method of tumor treatment is to improve or reshape the TME, but during the treatment process, TME is a dynamic process with close relationships between components. For example, CAFs can change the structure and hardness of ECM in various ways, and TGF- β Affects multiple immune cells. A deep understanding of the composition of TME and the connections between its components can contribute to the discovery of new targets and the development of methods for tumor treatment.
NPs have unique advantages in tumor treatment. NPs can passively target the tumor site through the EPR effect, and a certain range of particle sizes is conducive to infiltration into the tumor interior and cell capture. Improve the solubility and bioavailability of drugs while reducing drug toxicity. However, it has been found that NPs are easily engulfed by RES as foreign substances after entering the human body, greatly reducing their anticancer efficacy. Therefore, simulating NPs as biomimetic nanoparticles with endogenous components has become a research direction for tumor treatment nanocarriers. Experiments have found that biomimetic nanocarriers can avoid the phagocytosis of RES, significantly improving drug delivery efficiency and efficacy. Therefore, this article focuses on introducing TME related biomimetic nanocarriers, including different biomimetic carriers derived from proteins, cell membranes, and natural ligands. Research has shown that biomimetic nanocarriers have significant advantages in targeting and avoiding immune system phagocytosis. In addition to delivering targeted drugs, biomimetic NPs can also be applied to optical imaging and tumor vaccines during cancer diagnosis.
Although biomimetic nanoparticles have made significant progress in preclinical research; however, bionic NPs still have some problems like complicated cell membrane extraction routes, poor reproducibility, easy protein degeneration in preparation and preservation, resulting in adverse reactions, unable to prove the integrity of the wrapped cell membrane through experiments, clinical transformation difficulties, such as: the anti-cancer efficacy and long-term safety of biomimetic nanoparticles have not yet been fully established in the human body, the extrusion method for preparing biomimetic nanoparticles is time-consuming, inefficient, and difficult to scale up in industrial production, etc. In addition, in order to develop multifunctional intelligent cell membrane encapsulated nanoparticles, it is essential to modify the cell membrane, but this increases the risk of causing side effects. More importantly, excessive use of immune cell membrane coated nanoparticles may induce or exacerbate inflammation. Although there are many problems to be solved, biomimetic NPs offer a novel idea for nano-delivery systems for tumor therapy and have a good prospect.
This work was supported by the National Science Foundation of China (No. 81573621), the Natural Science Foundation of Hunan Province (No. 2022JJ40320), the Science and Technology Innovation Program of Hunan Province (2021RC4064), Key Discipline Project on Chinese Pharmacology of Hunan University of Chinese Medicine (202302), Excellent Youth Project of Hunan Education Department (23B0362), and Key Research and Development Plan of Hunan Province (No. 2017SK2122).
Lili Zhou and Cheng Peng drafted the manuscript. Yilin Xu, Jing Wu and Donghai Wu collected important background information and translated manuscripts. Xinhua Xia reviewed and revised manuscripts. All authors contributed to data analysis, drafting or revising the article, have agreed on the journal to which the article will be submitted, gave final approval of the version to be published, and agree to be accountable for all aspects of the work.
The authors report no conflicts of interest in this work.
1. Brunetto E, De Monte L, Balzano G, et al. The IL-1/IL-1 receptor axis and tumor cell released inflammasome adaptor ASC are key regulators of TSLP secretion by cancer associated fibroblasts in pancreatic cancer. J Immunother Cancer. 2019;7(1):45. doi:10.1186/s40425-019-0521-4
2. Melisi D, Garcia-Carbonero R, Macarulla T, et al. Galunisertib plus gemcitabine vs. gemcitabine for first-line treatment of patients with unresectable pancreatic cancer. Br J Cancer. 2018;119(10):1208–1214. doi:10.1038/s41416-018-0246-z
3. Coulson R, Liew SH, Connelly AA, et al. The angiotensin receptor blocker, Losartan, inhibits mammary tumor development and progression to invasive carcinoma. Oncotarget. 2017;8(12):18640–18656. doi:10.18632/oncotarget.15553
4. Jabłońska-Trypuć A, Matejczyk M, Rosochacki S. Matrix metalloproteinases (MMPs), the main extracellular matrix (ECM) enzymes in collagen degradation, as a target for anticancer drugs. J Enzyme Inhib Med Chem. 2016;31(sup1):177–183. doi:10.3109/14756366.2016.1161620
5. Dinca SC, Greiner D, Weidenfeld K, Bond L, Barkan D, and Jorcyk CL. Novel mechanism for OSM-promoted extracellular matrix remodeling in breast cancer: LOXL2 upregulation and subsequent ECM alignment. Breast Cancer Res. 2021;23(1):56. doi:10.1186/s13058-021-01430-x
6. De Vita A, Liverani C, Molinaro R, et al. Lysyl oxidase engineered lipid nanovesicles for the treatment of triple negative breast cancer. Sci Rep. 2021;11(1):5107. doi:10.1038/s41598-021-84492-3
7. Röhrig F, Vorlová S, Hoffmann H, et al. VEGF-ablation therapy reduces drug delivery and therapeutic response in ECM-dense tumors. Oncogene. 2017;36(1):1–12. doi:10.1038/onc.2016.182
8. Eatmann AI, Hamouda E, Hamouda H, et al. Potential use of thalidomide in glioblastoma treatment: an updated brief overview. Metabolites. 2023;13(4):543. doi:10.3390/metabo13040543
9. Naderi H, Matin MM, Bahrami AR. Review paper: critical issues in tissue engineering: biomaterials, cell sources, angiogenesis, and drug delivery systems. J Biomater Appl. 2011;26(4):383–417. doi:10.1177/0885328211408946
10. Beltrán-Partida E, Valdez-Salas B, García-López Portillo M, et al. Atherosclerotic-derived endothelial cell response conducted by titanium oxide nanotubes. Materials. 2023;16(2):794. doi:10.3390/ma16020794
11. Kim GH, Won JE, Byeon Y, et al. Selective delivery of PLXDC1 small interfering RNA to endothelial cells for anti-angiogenesis tumor therapy using CD44-targeted chitosan nanoparticles for epithelial ovarian cancer. Drug Deliv. 2018;25(1):1394–1402. doi:10.1080/10717544.2018.1480672
12. Lv J, Wang S, Qiao D, Lin Y, Hu S, and Li M. Mitochondria-targeting multifunctional nanoplatform for cascade phototherapy and hypoxia-activated chemotherapy. J Nanobiotechnology. 2022;20(1):42. doi:10.1186/s12951-022-01244-9
13. Yang X, Zhao M, Wu Z, et al. Nano-ultrasonic contrast agent for chemoimmunotherapy of breast cancer by immune metabolism reprogramming and tumor autophagy. ACS Nano. 2022;16(2):3417–3431. doi:10.1021/acsnano.2c00462
14. Li X, Pan J, Li Y, et al. Development of a localized drug delivery system with a step-by-step cell internalization capacity for cancer immunotherapy. ACS Nano. 2022;16(4):5778–5794. doi:10.1021/acsnano.1c10892
15. Li Z, Ding Y, Liu J, et al. Depletion of tumor associated macrophages enhances local and systemic platelet-mediated anti-PD-1 delivery for post-surgery tumor recurrence treatment. Nat Commun. 2022;13(1):1845. doi:10.1038/s41467-022-29388-0
16. Weber ANR, Bittner Z, Liu X, Dang TM, Radsak MP, and Brunner C. Bruton’s tyrosine kinase: an emerging key player in innate immunity. Front Immunol. 2017;8:1454. doi:10.3389/fimmu.2017.01454
17. Wang X, Ye L, He W, et al. In situ targeting nanoparticles-hydrogel hybrid system for combined chemo-immunotherapy of glioma. J Control Release. 2022;345:786–797. doi:10.1016/j.jconrel.2022.03.050
18. Lee JA, Shin JM, Song SH, et al. Recruitment of dendritic cells using ‘find-me’ signaling microparticles for personalized cancer immunotherapy. Biomaterials. 2022;282:121412. doi:10.1016/j.biomaterials.2022.121412
19. Wang W, Xu H, Ye Q, et al. Systemic immune responses to irradiated tumours via the transport of antigens to the tumour periphery by injected flagellate bacteria. Nat Biomed Eng. 2022;6(1):44–53. doi:10.1038/s41551-021-00834-6
20. Yao H, Shen N, Ji G, et al. Cisplatin nanoparticles promote intratumoral CD8(+) T cell priming via antigen presentation and t cell receptor crosstalk. Nano Lett. 2022;22(8):3328–3339. doi:10.1021/acs.nanolett.2c00478
21. Liu Y, Wang Y, Yang Y, et al. Emerging phagocytosis checkpoints in cancer immunotherapy. Signal Transduct Target Ther. 2023;8(1):104. doi:10.1038/s41392-023-01365-z
22. Kim J, Kang S, Kim KW, et al. Nanoparticle delivery of recombinant IL-2 (BALLkine-2) achieves durable tumor control with less systemic adverse effects in cancer immunotherapy. Biomaterials. 2022;280:121257. doi:10.1016/j.biomaterials.2021.121257
23. Pei L, Liu Y, Liu L, et al. Roles of cancer-associated fibroblasts (CAFs) in anti- PD-1/PD-L1 immunotherapy for solid cancers. Mol Cancer. 2023;22(1):29. doi:10.1186/s12943-023-01731-z
24. Linares J, Marín-Jiménez JA, Badia-Ramentol J, and Calon A. Determinants and Functions of CAFs secretome during cancer progression and therapy. Front Cell Dev Biol. 2020;8:621070. doi:10.3389/fcell.2020.621070
25. Komohara Y, Takeya M. CAFs and TAMs: maestros of the tumour microenvironment. J Pathol. 2017;241(3):313–315. doi:10.1002/path.4824
26. Ding Z, Shi R, Hu W, et al. Cancer-associated fibroblasts in hematologic malignancies: elucidating roles and spotlighting therapeutic targets. Front Oncol. 2023;13:1193978. doi:10.3389/fonc.2023.1193978
27. Saw PE, Chen J, Song E. Targeting CAFs to overcome anticancer therapeutic resistance. Trends Cancer. 2022;8(7):527–555. doi:10.1016/j.trecan.2022.03.001
28. Geng X, Chen H, Zhao L, et al. Cancer-Associated Fibroblast (CAF) heterogeneity and targeting therapy of CAFs in pancreatic cancer. Front Cell Dev Biol. 2021;9:655152. doi:10.3389/fcell.2021.655152
29. De P, Aske J, Sulaiman R, Dey N. Bête noire of chemotherapy and targeted therapy: CAF-Mediated resistance. Cancers. 2022;14(6). doi:10.3390/cancers14061519
30. Barcus CE, Hwang PY, Morikis V, et al. Tyrosine kinase-independent actions of DDR2 in tumor cells and cancer-associated fibroblasts influence tumor invasion, migration and metastasis. J Cell Sci. 2021;134(19). doi:10.1242/jcs.258431
31. Anderson NM, Simon MC. The tumor microenvironment. Curr Biol. 2020;30(16):R921–R925. doi:10.1016/j.cub.2020.06.081
32. Arneth B. Tumor Microenvironment. Medicina. 2019;56(1). doi:10.3390/medicina56010015
33. Seewaldt V. ECM stiffness paves the way for tumor cells. Nat Med. 2014;20(4):332–333. doi:10.1038/nm.3523
34. Graf F, Horn P, Ho AD, Boutros M, and Maercker C. The extracellular matrix proteins type I collagen, type III collagen, fibronectin, and laminin 421 stimulate migration of cancer cells. FASEB j. 2021;35(7):e21692. doi:10.1096/fj.202002558RR
35. Di Martino JS, Akhter T, Bravo-Cordero JJ. Remodeling the ECM: implications for metastasis and tumor dormancy. Cancers. 2021;13(19):4916. doi:10.3390/cancers13194916
36. Bao Y, Wang L, Shi L, et al. Transcriptome profiling revealed multiple genes and ECM-receptor interaction pathways that may be associated with breast cancer. Cell Mol Biol Lett. 2019;24:38. doi:10.1186/s11658-019-0162-0
37. Schedin P, Keely PJ. Mammary gland ECM remodeling, stiffness, and mechanosignaling in normal development and tumor progression. Cold Spring Harb Perspect Biol. 2011;3(1):a003228–a003228. doi:10.1101/cshperspect.a003228
38. Sun W. Angiogenesis in metastatic colorectal cancer and the benefits of targeted therapy. J Hematol Oncol. 2012;5:63. doi:10.1186/1756-8722-5-63
39. Park J, Lee H, Tran Q, et al. Recognition of transmembrane protein 39A as a tumor-specific marker in brain tumor. Toxicol Res. 2017;33(1):63–69. doi:10.5487/TR.2017.33.1.063
40. Sánchez Ramírez J, Morera Díaz Y, Bequet-Romero M, et al. Specific humoral response in cancer patients treated with a VEGF-specific active immunotherapy procedure within a compassionate use program. BMC Immunol. 2020;21(1):12. doi:10.1186/s12865-020-0338-4
41. Thomas D, Radhakrishnan P. Tumor-stromal crosstalk in pancreatic cancer and tissue fibrosis. Mol Cancer. 2019;18(1):14. doi:10.1186/s12943-018-0927-5
42. Kim MY, Shin JY, Kim JO, et al. Anti-tumor efficacy of CKD-516 in combination with radiation in xenograft mouse model of lung squamous cell carcinoma. BMC Cancer. 2020;20(1):1057. doi:10.1186/s12885-020-07566-x
43. Carson-Walter EB, Winans BN, Whiteman MC, et al. Characterization of TEM1/endosialin in human and murine brain tumors. BMC Cancer. 2009;9:417. doi:10.1186/1471-2407-9-417
44. Lee N, Yoo D, Ling D, Cho MH, Hyeon T, and Cheon J. Iron oxide based nanoparticles for multimodal imaging and magnetoresponsive therapy. Chem Rev. 2015;115(19):10637–10689. doi:10.1021/acs.chemrev.5b00112
45. Joyce CE, Zhou X, Xia J, et al. Deep sequencing of small RNAs from human skin reveals major alterations in the psoriasis miRNAome. Hum Mol Genet. 2011;20(20):4025–4040. doi:10.1093/hmg/ddr331
46. Choi YS, Jang H, Gupta B, et al. Tie2-mediated vascular remodeling by ferritin-based protein C nanoparticles confers antitumor and anti-metastatic activities. J Hematol Oncol. 2020;13(1):123. doi:10.1186/s13045-020-00952-9
47. Emoto M, Naganuma Y, Choijamts B, et al. Novel chemoembolization using calcium-phosphate ceramic microsphere incorporating TNP-470, an anti-angiogenic agent. Cancer Sci. 2010;101(4):984–990. doi:10.1111/j.1349-7006.2009.01479.x
48. Hossain MA, Liu G, Dai B, et al. Reinvigorating exhausted CD8(+) cytotoxic T lymphocytes in the tumor microenvironment and current strategies in cancer immunotherapy. Med Res Rev. 2021;41(1):156–201. doi:10.1002/med.21727
49. Bejarano L, Jordāo MJC, Joyce JA. Therapeutic targeting of the tumor microenvironment. Cancer Discov. 2021;11(4):933–959. doi:10.1158/2159-8290.CD-20-1808
50. Tsai MJ, Chang WA, Huang MS, and Kuo PL. Tumor microenvironment: a new treatment target for cancer. ISRN Biochem. 2014;2014:351959. doi:10.1155/2014/351959
51. Xiao Y, Yu D. Tumor microenvironment as a therapeutic target in cancer. Pharmacol Ther. 2021;221:107753. doi:10.1016/j.pharmthera.2020.107753
52. Gocher AM, Workman CJ, Vignali DAA. Interferon-γ: teammate or opponent in the tumour microenvironment? Nat Rev Immunol. 2022;22(3):158–172. doi:10.1038/s41577-021-00566-3
53. Pagano E, Elias JE, Schneditz G, et al. Activation of the GPR35 pathway drives angiogenesis in the tumour microenvironment. Gut. 2022;71(3):509–520. doi:10.1136/gutjnl-2020-323363
54. Borriello L, Coste A, Traub B, et al. Primary tumor associated macrophages activate programs of invasion and dormancy in disseminating tumor cells. Nat Commun. 2022;13(1):626. doi:10.1038/s41467-022-28076-3
55. Yang M, Li J, Gu P, and Fan X. The application of nanoparticles in cancer immunotherapy: targeting tumor microenvironment. Bioact Mater. 2021;6(7):1973–1987. doi:10.1016/j.bioactmat.2020.12.010
56. Müller AK, Köhler UA, Trzebanski S, et al. Mouse modeling dissecting macrophage-breast cancer communication uncovered roles of PYK2 in macrophage recruitment and breast tumorigenesis. Adv Sci. 2022;9(9):e2105696. doi:10.1002/advs.202105696
57. Hu M, Huang L. Strategies targeting tumor immune and stromal microenvironment and their clinical relevance. Adv Drug Deliv Rev. 2022;183:114137. doi:10.1016/j.addr.2022.114137
58. Guo R, Wang S, Zhao L, et al. Engineered nanomaterials for synergistic photo-immunotherapy. Biomaterials. 2022;282:121425. doi:10.1016/j.biomaterials.2022.121425
59. Huang TX, Tan XY, Huang HS, et al. Targeting cancer-associated fibroblast-secreted WNT2 restores dendritic cell-mediated antitumour immunity. Gut. 2022;71(2):333–344. doi:10.1136/gutjnl-2020-322924
60. Cheung PF, Yang J, Fang R, et al. Progranulin mediates immune evasion of pancreatic ductal adenocarcinoma through regulation of MHCI expression. Nat Commun. 2022;13(1):156. doi:10.1038/s41467-021-27088-9
61. Duong E, Fessenden TB, Lutz E, et al. Type I interferon activates MHC class I-dressed CD11b+ conventional dendritic cells to promote protective anti-tumor CD8+ T cell immunity. Immunity. 2022;55(2):308–323.e309. doi:10.1016/j.immuni.2021.10.020
62. Srinivasan S, Kryza T, Batra J, and Clements J. Remodelling of the tumour microenvironment by the kallikrein-related peptidases. Nat Rev Cancer. 2022;22(4):223–238. doi:10.1038/s41568-021-00436-z
63. Downs-Canner SM, Meier J, Vincent BG, and Serody JS. B cell function in the tumor microenvironment. Annu Rev Immunol. 2022;40:169–193. doi:10.1146/annurev-immunol-101220-015603
64. Kang JH, Zappasodi R. Modulating Treg stability to improve cancer immunotherapy. Trends Cancer. 2023;9(11):911–927. doi:10.1016/j.trecan.2023.07.015
65. Tauriello DVF, Sancho E, Batlle E. Overcoming TGFβ-mediated immune evasion in cancer. Nat Rev Cancer. 2022;22(1):25–44. doi:10.1038/s41568-021-00413-6
66. Gavrielatou N, Vathiotis I, Economopoulou P, and Psyrri A. The role of B cells in head and neck cancer. Cancers. 2021;13(21):5383. doi:10.3390/cancers13215383
67. Biber G, Sabag B, Raiff A, et al. Modulation of intrinsic inhibitory checkpoints using nano-carriers to unleash NK cell activity. EMBO Mol Med. 2022;14(1):e14073. doi:10.15252/emmm.202114073
68. Hu W, Wang G, Huang D, Sui M, and Xu Y. Cancer immunotherapy based on natural killer cells: current progress and new opportunities. Front Immunol. 2019;10:1205. doi:10.3389/fimmu.2019.01205
69. Tong L, Jiménez-Cortegana C, Tay AHM, Wickström S, Galluzzi L, and Lundqvist A. NK cells and solid tumors: therapeutic potential and persisting obstacles. Mol Cancer. 2022;21(1):206. doi:10.1186/s12943-022-01672-z
70. Liu H, Wang Z, Zhou Y, and Yang Y. MDSCs in breast cancer: an important enabler of tumor progression and an emerging therapeutic target. Front Immunol. 2023;14:1199273. doi:10.3389/fimmu.2023.1199273
71. Li K, Shi H, Zhang B, et al. Myeloid-derived suppressor cells as immunosuppressive regulators and therapeutic targets in cancer. Signal Transduct Target Ther. 2021;6(1):362. doi:10.1038/s41392-021-00670-9
72. Hei Y, Chen Y, Li Q, et al. Multifunctional immunoliposomes enhance the immunotherapeutic effects of PD-L1 antibodies against melanoma by reprogramming immunosuppressive tumor microenvironment. Small. 2022;18(9):e2105118. doi:10.1002/smll.202105118
73. Zhai Q, Chen Y, Xu J, et al. Lymphoma immunochemotherapy: targeted delivery of doxorubicin via a dual functional nanocarrier. Mol Pharm. 2017;14(11):3888–3895. doi:10.1021/acs.molpharmaceut.7b00606
[ PMC free article ] [ PubMed ] 74. Rattan R, Bhattacharjee S, Zong H, et al.Nanoparticle-macrophage interactions: a balance between clearance and cell-specific targeting.Bioorg Med Chem.2017;25(16):4487–4496.doi:10.1016/j.bmc.2017.06.040
75. Fang RH, Jiang Y, Fang JC, and Zhang L. Cell membrane-derived nanomaterials for biomedical applications. Biomaterials. 2017;128:69–83. doi:10.1016/j.biomaterials.2017.02.041
76. Vandchali NR, Moadab F, Taghizadeh E, Tajbakhsh A, and Gheibihayat SM. CD47 Functionalization of Nanoparticles as a Poly(ethylene glycol) alternative: a novel approach to improve drug delivery. Curr Drug Targets. 2021;22(15):1750–1759. doi:10.2174/1389450122666210204203514
77. Jalil AR, Tobin MP, Discher DE. Suppressing or enhancing macrophage engulfment through the use of CD47 and related peptides. Bioconjug Chem. 2022;33(11):1989–1995. doi:10.1021/acs.bioconjchem.2c00019
78. Hu CM, Zhang L, Aryal S, Cheung C, Fang RH, and Zhang L. Erythrocyte membrane-camouflaged polymeric nanoparticles as a biomimetic delivery platform. Proc Natl Acad Sci U S A. 2011;108(27):10980–10985. doi:10.1073/pnas.1106634108
79. Gheibi Hayat SM, Bianconi V, Pirro M, and Sahebkar A. Stealth functionalization of biomaterials and nanoparticles by CD47 mimicry. Int J Pharm. 2019;569:118628. doi:10.1016/j.ijpharm.2019.118628
80. Pei Q, Hu X, Zheng X, et al. Light-activatable red blood cell membrane-camouflaged dimeric prodrug nanoparticles for synergistic photodynamic/chemotherapy. ACS Nano. 2018;12(2):1630–1641. doi:10.1021/acsnano.7b08219
81. Liu H, Su YY, Jiang XC, and Gao JQ. Cell membrane-coated nanoparticles: a novel multifunctional biomimetic drug delivery system. Drug Deliv Transl Res. 2023;13(3):716–737. doi:10.1007/s13346-022-01252-0
82. Han Y, Gao C, Wang H, et al.Corrigendum to ”Macrophage membrane-coated nanocarriers Co-Modified by RVG29 and TPP improve brain neuronal mitochondria-targeting and therapeutic efficacy in Alzheimer’s disease mice. Bioact Mater. 2022;(7):73. doi:10.1016/j.bioactmat.2021.06.002
83. Hu T, Huang Y, Liu J, Shen C, Wu F, and He Z. Biomimetic cell-derived nanoparticles: emerging platforms for cancer immunotherapy. Pharmaceutics. 2023;15(7):1821. doi:10.3390/pharmaceutics15071821
84. Fang RH, Hu CM, Luk BT, et al. Cancer cell membrane-coated nanoparticles for anticancer vaccination and drug delivery. Nano Lett. 2014;14(4):2181–2188. doi:10.1021/nl500618u
[ PMC free article ] [ PubMed ] 85. Wu Z, Zhang H, Yan J, Wei Y, and Su J. Engineered biomembrane-derived nanoparticles for nanoscale theranostics.Theranostics.2023;13(1):20–39.doi:10.7150/thno.76894
86. Li S, Wu Y, Ding F, et al. Engineering macrophage-derived exosomes for targeted chemotherapy of triple-negative breast cancer. Nanoscale. 2020;12(19):10854–10862. doi:10.1039/D0NR00523A
87. Zhang X, Zhang H, Gu J, et al. Engineered extracellular vesicles for cancer therapy. Adv Mater. 2021;33:14.
88. Smyth T, Kullberg M, Malik N, Smith-Jones P, Graner MW, and Anchordoquy TJ. Biodistribution and delivery efficiency of unmodified tumor-derived exosomes. J Control Release. 2015;199:145–155. doi:10.1016/j.jconrel.2014.12.013
[ PMC free article ] [ PubMed ] 89. Xu M, Feng T, Liu B, et al.Engineered exosomes: desirable target-tracking characteristics for cerebrovascular and neurodegenerative disease therapies.Theranostics.2021;11(18):8926–8944.doi:10.7150/thno.62330
90. Zhang M, Hu S, Liu L, et al. Engineered exosomes from different sources for cancer-targeted therapy. Signal Transduct Target Ther. 2023;8(1):124. doi:10.1038/s41392-023-01382-y
91. Xie H, Li W, Liu H, et al. Erythrocyte membrane-coated invisible acoustic-sensitive nanoparticle for inducing tumor thrombotic infarction by precisely damaging tumor vascular endothelium. Small. 2022;18(30):e2201933. doi:10.1002/smll.202201933
92. Rao L, Bu LL, Xu JH, et al. Red blood cell membrane as a biomimetic nanocoating for prolonged circulation time and reduced accelerated blood clearance. Small. 2015;11(46):6225–6236. doi:10.1002/smll.201502388
93. Liu T, Shi C, Duan L, et al. A highly hemocompatible erythrocyte membrane-coated ultrasmall selenium nanosystem for simultaneous cancer radiosensitization and precise antiangiogenesis. J Mater Chem B. 2018;6(29):4756–4764. doi:10.1039/C8TB01398E
94. Liang X, Ye X, Wang C, et al. Photothermal cancer immunotherapy by erythrocyte membrane-coated black phosphorus formulation. J Control Release. 2019;296:150–161. doi:10.1016/j.jconrel.2019.01.027
95. Ren H, Liu J, Li Y, et al. Oxygen self-enriched nanoparticles functionalized with erythrocyte membranes for long circulation and enhanced phototherapy. Acta Biomater. 2017;59:269–282. doi:10.1016/j.actbio.2017.06.035
96. Chen Q, Zhang L, Li L, et al. Cancer cell membrane-coated nanoparticles for bimodal imaging-guided photothermal therapy and docetaxel-enhanced immunotherapy against cancer. J Nanobiotechnology. 2021;19(1):449. doi:10.1186/s12951-021-01202-x
97. Park W, Seong KY, Han HH, Yang SY, and Hahn SK. Dissolving microneedles delivering cancer cell membrane coated nanoparticles for cancer immunotherapy. RSC Adv. 2021;11(17):10393–10399. doi:10.1039/D1RA00747E
98. Gan J, Du G, He C, et al. Tumor cell membrane enveloped aluminum phosphate nanoparticles for enhanced cancer vaccination. J Control Release. 2020;326:297–309. doi:10.1016/j.jconrel.2020.07.008
99. Li Z, Cai H, Li Z, et al. A tumor cell membrane-coated self-amplified nanosystem as a nanovaccine to boost the therapeutic effect of anti-PD-L1 antibody. Bioact Mater. 2023;21:299–312. doi:10.1016/j.bioactmat.2022.08.028
100. Jin J, Krishnamachary B, Barnett JD, et al. Human cancer cell membrane-coated biomimetic nanoparticles reduce fibroblast-mediated invasion and metastasis and induce T-cells. ACS Appl Mater Interfaces. 2019;11(8):7850–7861. doi:10.1021/acsami.8b22309
101. Molinaro R, Martinez JO, Zinger A, et al. Leukocyte-mimicking nanovesicles for effective doxorubicin delivery to treat breast cancer and melanoma. Biomater Sci. 2020;8(1):333–341. doi:10.1039/C9BM01766F
102. Chen C, Song M, Du Y, et al. Tumor-associated-macrophage-membrane-coated nanoparticles for improved photodynamic immunotherapy. Nano Lett. 2021;21(13):5522–5531. doi:10.1021/acs.nanolett.1c00818
103. Cao X, Tan T, Zhu D, et al. Paclitaxel-loaded macrophage membrane camouflaged albumin nanoparticles for targeted cancer therapy. Int J Nanomed. 2020;15:1915–1928. doi:10.2147/IJN.S244849
104. Huang X, Wang L, Guo H, and Zhang W. Macrophage membrane-coated nanovesicles for dual-targeted drug delivery to inhibit tumor and induce macrophage polarization. Bioact Mater. 2023;23:69–79. doi:10.1016/j.bioactmat.2022.09.027
105. Yin T, Fan Q, Hu F, et al. Engineered macrophage-membrane-coated nanoparticles with enhanced PD-1 expression induce immunomodulation for a synergistic and targeted antiglioblastoma activity. Nano Lett. 2022;22(16):6606–6614. doi:10.1021/acs.nanolett.2c01863
106. Pitchaimani A, Nguyen TDT, Aryal S. Natural killer cell membrane infused biomimetic liposomes for targeted tumor therapy. Biomaterials. 2018;160:124–137. doi:10.1016/j.biomaterials.2018.01.018
107. Cao X, Hu Y, Luo S, et al. Neutrophil-mimicking therapeutic nanoparticles for targeted chemotherapy of pancreatic carcinoma. Acta Pharm Sin B. 2019;9(3):575–589. doi:10.1016/j.apsb.2018.12.009
108. Zhao J, Lu H, Xu D, et al. Neutrophil membrane-coated nanoparticles for enhanced nanosecond pulsed electric field treatment of pancreatic cancer. Int J Hyperthermia. 2022;39(1):1026–1035. doi:10.1080/02656736.2022.2093994
109. Wang H, Wu J, Williams GR, et al. Platelet-membrane-biomimetic nanoparticles for targeted antitumor drug delivery. J Nanobiotechnology. 2019;17(1):60. doi:10.1186/s12951-019-0494-y
110. Jiang Q, Wang K, Zhang X, et al. Platelet membrane‐camouflaged magnetic nanoparticles for ferroptosis‐enhanced cancer immunotherapy. Small. 2020;16(22):e2001704. doi: 10.1002/smll.202001704
111. Wang C, Sun W, Ye Y, Hu Q, Bomba HN, and Gu Z. In situ activation of platelets with checkpoint inhibitors for post-surgical cancer immunotherapy. Nat Biomed Eng. 2017;1(2):0011. doi:10.1038/s41551-016-0011
112. Li B, Chu T, Wei J, et al. Platelet-membrane-coated nanoparticles enable vascular disrupting agent combining anti-angiogenic drug for improved tumor vessel impairment. Nano Lett. 2021;21(6):2588–2595. doi:10.1021/acs.nanolett.1c00168
113. Luo X, Cao J, Yu J, et al. Regulating acidosis and relieving hypoxia by platelet membrane-coated nanoparticle for enhancing tumor chemotherapy. Front Bioeng Biotechnol. 2022;10:885105. doi:10.3389/fbioe.2022.885105
114. Dehaini D, Wei X, Fang RH, et al. Erythrocyte-platelet hybrid membrane coating for enhanced nanoparticle functionalization. Adv Mater. 2017;29(16). doi:10.1002/adma.201606209
115. Gong C, Yu X, Zhang W, et al. Regulating the immunosuppressive tumor microenvironment to enhance breast cancer immunotherapy using pH-responsive hybrid membrane-coated nanoparticles. J Nanobiotechnology. 2021;19(1):58. doi:10.1186/s12951-021-00805-8
116. Gong C, Yu X, You B, et al. Macrophage-cancer hybrid membrane-coated nanoparticles for targeting lung metastasis in breast cancer therapy. J Nanobiotechnology. 2020;18(1):92. doi:10.1186/s12951-020-00649-8
117. Wang Y, Luan Z, Zhao C, Bai C, and Yang K. Target delivery selective CSF-1R inhibitor to tumor-associated macrophages via erythrocyte-cancer cell hybrid membrane camouflaged pH-responsive copolymer micelle for cancer immunotherapy. Eur J Pharm Sci. 2020;142:105136. doi:10.1016/j.ejps.2019.105136
118. Feng J, Xiang L, Fang C, et al. Dual-targeting of tumor cells and tumor-associated macrophages by palmitic acid modified albumin nanoparticles for antitumor and antimetastasis therapy. ACS Appl Mater Interfaces. 2022;14(13):14887–14902. doi:10.1021/acsami.1c23274
119. Wang X, Liu Y, Xue C, et al. A protein-based cGAS-STING nanoagonist enhances T cell-mediated anti-tumor immune responses. Nat Commun. 2022;13(1):5685. doi:10.1038/s41467-022-33301-0
120. Rabie EM, Zhang SX, Kourouklis AP, et al. Matrix degradation and cell proliferation are coupled to promote invasion and escape from an engineered human breast microtumor. Integr Biol. 2021;13(1):17–29. doi:10.1093/intbio/zyaa026
121. Chen B, Dong X, Dong X, et al. Integration of dual targeting and dual therapeutic modules endows self-assembled nanoparticles with anti-tumor growth and metastasis functions. Int J Nanomed. 2021;16:1361–1376. doi:10.2147/IJN.S291285
122. Mine Y, Munir H, Nakanishi Y, and Sugiyama D. Biomimetic peptides for the treatment of cancer. Anticancer Res. 2016;36(7):3565–3570.
123. Wang S, Blois A, El Rayes T, et al. Development of a prosaposin-derived therapeutic cyclic peptide that targets ovarian cancer via the tumor microenvironment. Sci Transl Med. 2016;8(329):329ra334. doi:10.1126/scitranslmed.aad5653
124. Xiang Y, Chen L, Zhou R, and Huang Y. Enhanced intracellular and intranuclear drug delivery mediated by biomimetic peptide SVS-1 for anticancer therapy. Int J Pharm. 2019;570:118668. doi:10.1016/j.ijpharm.2019.118668
125. Qing S, Lyu C, Zhu L, et al. Biomineralized bacterial outer membrane vesicles potentiate safe and efficient tumor microenvironment reprogramming for anticancer therapy. Adv Mater. 2020;32:47.
126. Nie W, Yu T, Liu X, et al. Non-viral vector mediated CKb11 with folic acid modification regulates macrophage polarization and DC maturation to elicit immune response against cancer. Bioact Mater. 2021;6(11):3678–3691. doi:10.1016/j.bioactmat.2021.03.031
127. Lu Y, Li L, Du J, et al. Immunotherapy for tumor metastasis by artificial antigen-presenting cells via targeted microenvironment regulation and T-Cell activation. ACS Appl Mater Interfaces. 2021;13(47):55890–55901. doi:10.1021/acsami.1c17498
128. Song Y, Xu M, Li Y, et al. An iRGD peptide fused superantigen mutant induced tumor-targeting and T lymphocyte infiltrating in cancer immunotherapy. Int J Pharm. 2020;586:119498. doi:10.1016/j.ijpharm.2020.119498
129. Kim M, Lee JS, Kim W, et al. Aptamer-conjugated nano-liposome for immunogenic chemotherapy with reversal of immunosuppression. J Control Release. 2022;348:893–910. doi:10.1016/j.jconrel.2022.06.039
130. Chen Q, Li Q, Liang Y, et al. Natural exosome-like nanovesicles from edible tea flowers suppress metastatic breast cancer via ROS generation and microbiota modulation. Acta Pharm Sin B. 2022;12(2):907–923. doi:10.1016/j.apsb.2021.08.016
131. Hu M, Zhang J, Kong L, et al. Immunogenic hybrid nanovesicles of liposomes and tumor-derived nanovesicles for cancer immunochemotherapy. ACS Nano. 2021;15(2):3123–3138. doi:10.1021/acsnano.0c09681
[ PubMed ] 132. Hu XX, He PP, Qi GB, et al.Transformable nanomaterials as an artificial extracellular matrix for inhibiting tumor invasion and metastasis.ACS Nano.2017;11(4):4086–4096.doi:10.1021/acsnano.7b00781
133. Zheng DW, Hong S, Zhang QL, et al. Controllable gelation of artificial extracellular matrix for altering mass transport and improving cancer therapies. Nat Commun. 2020;11(1):4907. doi:10.1038/s41467-020-18493-7
134. Vairavel M, Devaraj E, Shanmugam R. An eco-friendly synthesis of Enterococcus sp.-mediated gold nanoparticle induces cytotoxicity in human colorectal cancer cells. Environ Sci Pollut Res Int. 2020;27(8):8166–8175. doi:10.1007/s11356-019-07511-x
135. Selvanesan BC, Chandra D, Quispe-Tintaya W, et al. Listeria delivers tetanus toxoid protein to pancreatic tumors and induces cancer cell death in mice. Sci Transl Med. 2022;14(637):eabc1600. doi:10.1126/scitranslmed.abc1600
© 2024 The Author(s). This work is published and licensed by Dove Medical Press Limited. The full terms of this license are available at https://www.dovepress.com/terms.php and incorporate the Creative Commons Attribution - Non Commercial (unported, v3.0) License. By accessing the work you hereby accept the Terms. Non-commercial uses of the work are permitted without any further permission from Dove Medical Press Limited, provided the work is properly attributed. For permission for commercial use of this work, please see paragraphs 4.2 and 5 of our Terms.
Contact Us • Privacy Policy • Associations & Partners • Testimonials • Terms & Conditions • Recommend this site • Cookies • Top
Contact Us • Privacy Policy
© Copyright 2024 • Dove Press Ltd • software development by maffey.com • Web Design by Adhesion
The opinions expressed in all articles published here are those of the specific author(s), and do not necessarily reflect the views of Dove Medical Press Ltd or any of its employees.

Fmoc-Gly-OH Dove Medical Press is part of Taylor & Francis Group, the Academic Publishing Division of Informa PLC Copyright 2017 Informa PLC. All rights reserved. This site is owned and operated by Informa PLC ( “Informa”) whose registered office is 5 Howick Place, London SW1P 1WG. Registered in England and Wales. Number 3099067. UK VAT Group: GB 365 4626 36